the Creative Commons Attribution 4.0 License.
the Creative Commons Attribution 4.0 License.
The formation of ionospheric physics – confluence of traditions and threads of continuity
Aitor Anduaga
This paper examines how ionospheric physics emerged as a research speciality in Britain, Germany, and the United States in the first four decades of the 20th century. It argues that the formation of this discipline can be viewed as the confluence of four deep-rooted traditions in which scientists and engineers transformed, from within, research areas connected to radio wave propagation and geomagnetism. These traditions include Cambridge school's mathematical physics, Göttingen's mathematical physics, laboratory-based experimental physics, and Humboldtian-style terrestrial physics. Although focused on ionospheric physics, the paper pursues the idea that a dynamic conception of scientific tradition will provide a new perspective for the study of geosciences history.
A relatively recent trend in historical scholarship has been to emphasise the role of physical sub-disciplines in the emergence of geophysics and geo- and space sciences. The field of ionospheric physics does not escape the net. As one finds out more about its formation in the interwar years, one becomes increasingly convinced that such a process was more the result of a reconceptualisation and expansion of existing objects of enquiry than of the key milestones held by standard accounts; that is, the discovery of ionospheric layers in the 1920s and the development of theories of radio wave propagation in the 1930s (Green, 1946; Kenrick and Pickard, 1930; Mimno, 1937; Tuska, 1944). Historians, such as C. Stewart Gillmor, who maintain the narrative of discoveries and key events, also seek its origins in the early efforts of geomagneticians and radio experimenters (Gillmor, 1975, 1982, 1986, 1994).
Yet it is difficult to assess the influence of existing physical sub-disciplines on the emerging speciality of ionospheric physics in the 1920s. What role, for example, did radio and electrical engineers play in developing vacuum tubes and piezo-electric crystal circuits? Or how did geomagneticians' early auroral studies foster ionospheric research? Modern scholarship has not yet definitively discarded the somewhat simplistic view that the discoveries of the ionospheric layers provoked the birth of ionospheric physics nor has it been able to distinguish between patterns of change (in scientific ideas separated by time and place) and threads of intellectual and social continuity.
Regarding the more general discipline of geophysics, researchers have long been aware that its formation was somewhat the result of a process of assembly from frameworks of consensus that took place between the 19th and 20th centuries. In analysing this formation, historian Gregory A. Good characterised it as a process of extrication and recombination rather than accretion of disconnected fragments. For Good (2000), 20th century geophysics cannot be viewed as the culmination of a teleological process but rather as the progressive transformation of a large framework of consensus with multiple levels of complexity that are increasingly evolving.
The purpose of this article is to trace the major threads of intellectual and social continuity among the patterns of change which occurred in ionospheric physics in Britain, Germany, and the United States in the four decades before World War II (WWII). I shall argue that the confluence of scientific traditions rather than the branching into, or occupation of, hitherto unexplored areas defined the new speciality. A total of four threads – Cambridge school's mathematical physics, Göttingen's mathematical physics, laboratory-based experimental physics, and Humboldtian-style terrestrial physics – will be singled out as the major confluent traditions during those years and at those places. Such a set of hypotheses thus intends to modify current scholarship in the following three ways: first, by identifying as the main units of analysis not the discoveries and theories but the scientific traditions; second, by going beyond the narratives of individual findings and innovations and by insisting, instead, on the small groups pursuing coherent research programmes in the same institutional context; and third, by hypothesising that ionospheric physics evolved because the investigators transformed, from within, areas of physics, engineering, and telecommunications and especially geomagnetism and radio wave propagation.
It might be thought that the geographically limited nature of the question itself – how several traditions converged in those countries – would limit the validity of the conclusions as inferences on the formation of ionospheric physics in general. But I would argue that the threads of continuity concern the traditions of the discipline itself, rather than those of the countries involved. In Britain, ionospheric research was conducted by both physicists and mathematicians in university departments and by radio engineers from the Marconi Company and the Radio Research Board, and then it was extended to some British dominions. In Germany, ionospheric research essentially included academic, industrial, and military centres, as was the case in Britain. In the US, it was pursued mainly in university departments of electrical engineering and applied physics and government and industrial laboratories (see Tables 1, 2, and 3).
The reader might be tempted to think that the traditions examined here will perhaps over-reflect particular British, German, and US circumstances, distorting the state of the question as a whole. However, I think that these limitations by no means undermine the validity of generalising the history from the state of ionospheric physics in those countries to its state in general. Those countries were the loci of radio science in the interwar years, with far more advanced research groups than the rest. Pre-WWII France, for example, was a minor player, and ionospheric studies and forecasts for radiocommunications only entered the scene during and after the war (Pestre, 1997). Japan, on the other hand, was another locus that followed the German model, concentrating research on physics and geomagnetism centres (Gillmor, 1997). Britain, Germany, and the United States, therefore, mirror the confluence of traditions that one finds in ionospheric physics in its entirety.
Table 1Ionospheric physics in Britain in the interwar years. Groups and major research topics.
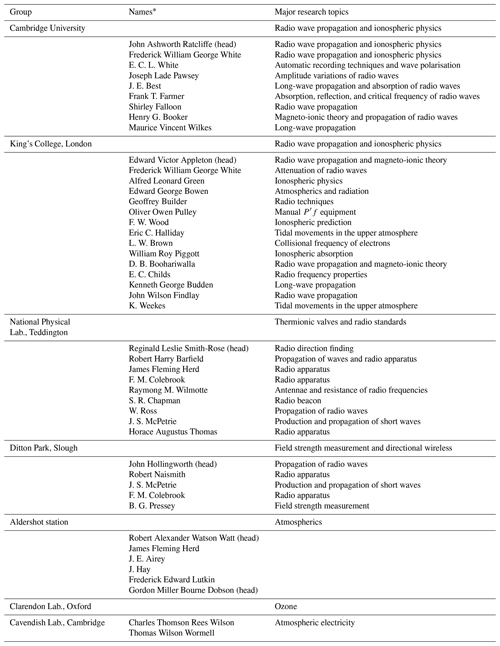
* The list of members is not exhaustive.
Table 2Ionospheric physics in the United States in the interwar years. Groups and major research topics.
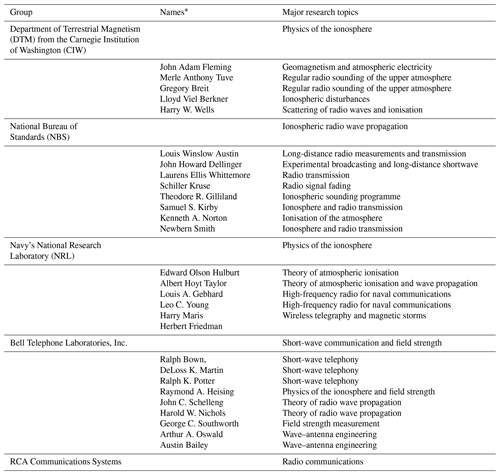
* The list of members is not exhaustive.
The construction of new proofs based upon axioms defines pure mathematics, while the application of mathematical methods defines mixed mathematics, which, in turn, defines applied mathematics and mathematical physics. The Cambridge Mathematical Tripos epitomises algebraic manipulative mastery with a physical reference (Warwick, 2003). The dozen graduates involved in this history all had vocational training for geophysical sciences and favoured the reception of electromagnetic theory, as expounded by James Clerk Maxwell. Their academic interests were predominantly focused on geomagnetism and the propagation of radio waves, and, to a lesser extent, on the ionisation of gases (Anduaga, 2016, p. 18; Wilson, 1982). For them, mathematics was inseparable from the physical problem to which it was applied. The atmosphere was a medium in which pure and applied mathematics coalesced into one single discipline. They all provide an excellent example of how mathematical physics was the route to understanding the nature and behaviour of the atmosphere.
In suggesting that the understanding of atmospheric nature was the highest priority of the Cambridge group, I am purposely making an undifferentiated statement. For, while the academic character and tradition of the group remained the same in the four decades or so up to World War II, their styles and research fields varied greatly. Throughout the 19th century, two approaches emerged in the field of geophysics in Britain. At Owens College, Manchester, Balfour Stewart conducted terrestrial magnetic investigations, while George Darwin constituted a group of physic-mathematical geology at Cambridge, specialising in the Earth's structure and crust (Kushner, 1993). However, from 1888, the character of geomagnetic studies began to change, albeit with no significant shift in the location of studies (from Owens College to the Victoria University of Manchester) when Cambridge physicist Arthur Schuster succeeded Stewart at Manchester and later established the New Physical Laboratory in 1900. In the 1900s, all that happened is that Schuster's geomagnetism school transcended the most routine forms of recording and collecting, distinctive of magnetic observatories (Kargon, 1977, pp. 220–237). His broad-mindedness in research topics, from geomagnetism to atmospheric dynamics and cosmic rays, made him something of a British paragon, meriting the mantle of respectability (Simpson, 1935; Lightman, 2004, pp. 1780–1784). The involvement of Sydney Chapman (a Manchester graduate in engineering and mathematics and then a Cambridge Wrangler at Trinity College) in frontier research projects on geomagnetism is entirely typical of the way in which Cambridge mixed mathematics approach broadened, rather than narrowed, geomagnetic studies (Anduaga, 2009b).
In addition to geomagnetic studies, Cambridge mathematicians explored the enigmatic phenomenon of transatlantic radiocommunication, i.e. the possibility of long-distance radio transmission in the atmosphere (Yeang, 2003, 2013; Anduaga, 2009a, pp. 4–6). Their propagation studies included diffraction rather than reflection theories. Unlike atmospheric–reflection experimentalists, diffraction theorists constructed mathematical representations based upon highly simplified atmospheric models. The way was paved by Hector Munro Macdonald in 1903. Macdonald formulated a surface diffraction theory to explain Marconi's transatlantic transmission, which was predicated not only on an over-idealised atmosphere (a free space with zero conductivity and uniform dielectric constant) but also on an image of the Earth (as a perfect conductor; Macdonald, 1903; Whittaker, 1935, pp. 553–555). Others – John William Nicholson and Augustus E. H. Love, in particular – essentially agreed with Macdonald's initial postulates, though they disagreed with how to approximate the analytical solution of diffracted field intensity (Nicholson, 1910; Love, 1915). So proficiently did they address the conundrum of diffracted field intensity that, for the 1900s and 1910s, diffraction theories became the sole theoretical alternative to the ionic refraction model proposed by William Henry Eccles in 1912.
From the start, long-distance radio wave propagation predominantly became the matter of two big communities, namely theorists and experimentalists. And it remained so for a long time, though Cambridge mathematicians became increasingly prominent among the former, and Anglo-American electrical engineers and experimental physicists set the pace among the latter, in the search for empirical quantitative relations. For theorists, the crux of the matter was to determine whether the waves travelling along the surface resulted from diffraction by the Earth or whether waves bounced back and forth between the Earth's surface and a reflecting/refracting layer. MacDonald, Nicholson, Love, and others propounded diffraction theories and succeeded in converting wave propagation fields into numerically tractable forms. But from 1918, when George Neville Watson, another Cambridge mathematician, demonstrated that surface diffraction alone could not explain empirical observations, the diffraction proponents' role began to wane (Watson, 1918a). Diffraction theories proved mathematically consistent, but their quantitative predictions did not match with the empirical law governing the relation between wave intensity and transmission distance – the so-called Austin-Cohen formula (Yeang, 2003). In the end, Watson applied the complex variable techniques to the model of a homogeneous and conducting boundary in order to obtain quantitative prediction that would be consistent with the empirical law (Watson, 1918, 1919a, b). Watson's attempt was in vain. Ironically, the last of the diffraction sagas paved the way for the rise of reflection theories in the 1920s (Whittaker, 1966, p. 523).
The origins of the Göttingen mathematical physics tradition go back to 1850 when the Hanoverian government established the Mathematics–Physics Seminar. According to its statutes, the centre was founded for the “training of teachers for mathematics and physics instruction at institutions of higher learning as well as the general elevation of the study of the mathematical and physical sciences” (Neuenschwander and Burmann, 1994, pp. 148–149). Felix Klein, a former professor of geometry at Leipzig, was its prime mover. Together with the legacy of weekly discussion meetings, scholarly rigour, and a sense of social responsibility, Klein brought along a sense of, and reverence for, seminar work that framed and regulated academic instruction. This was necessary for him to reach his main objective, namely the reinforcement of links between mathematics, the natural sciences, and industry (Klein, 1896). The combination of mathematics and economics is also revealed in his approach to institutional science. Under the aegis of main industrialists, in 1898 he founded the Göttingen Association for the Advancement of Applied Physics and Mathematics, which promoted the creation of numerous institutes – among them, those for Applied Electricity, Applied Mathematics and Mechanics, and the “Institut für Geophysik” (Institute of Geophysics).
Indeed, it was mathematics through useful arts (those concerned with practical subjects such as manufacture and craftsmanship) that defined Göttingen's interrelationship between mathematics and economics. Whereas the Cambridge Wranglers had sporadic contact with experimenters, Göttingen theorists had close links with engineers and experimental physicists. Few fostered the use of mathematics to application in the spirit of Klein as his assistant Arnold Sommerfeld did. Sommerfeld was a talented mathematical physicist who obtained the doctorate at Königsberg, the cradle of seminar method and of the unity of theory and instrumentation (Olesko, 1991). As Sommerfeld himself recognised, “Klein's programme for applying analytical mechanics, and higher mathematics generally, to engineering problems promised manifold mutual advantages” (Forman and Hermann, 1975, p. 526). When he became Privatdozent (private lecturer) in mathematics in 1895, his battle was determining how to reduce a mathematical physics problem into the evaluation of a complex integral. Together with other brilliant lecturers (David Hilbert, Hermann Minkowski, Edmund Landau, etc.), Göttingen would soon excel as “the seat of mathematical high culture” (Forman and Hermann, 1975, p. 526).
It was mathematics through useful arts that indeed made addressing the question of radio wave propagation possible in Germany, and paradoxically, the diffraction theories started to become engineering at the same time (Yeang, 2013, pp. 37–50). In 1906, Sommerfeld joined the chair of theoretical physics at the Ludwig Maximilian University of Munich, where he built a theoretical laboratory by emulating experimentalists' style, i.e. by encouraging students to conduct teamwork theoretical research (Jungnickel and McCormmach, 1990, pp. 157–160 and 277–285). Stimulated by his contact with leading wireless engineers (among them, Jonathan Zenneck, as shown below), he attributed the problem of radio wave propagation in the influence of ground conductivity to transmission (Eckert and Märker, 2000, p. 285). In contrast to Cambridge theorists, who only examined the influence of the geometric shape of the boundary surface, Sommerfeld adopted an integral approach to show how the resistance of the ground affected propagation distance (Sommerfeld, 1909, 1968). His approach was a great inspiration among his doctorate students, especially Herman William March and Witold von Rybczynski. A comparison of their dissertations from 1911 and 1913, respectively, makes the point very plain. In both cases, the focus on ground conductivity was united with the extension of Sommerfeld's integral approach to the diffracted waves along a spherical conductor. This implied unmistakably that there, at Munich, something resembling a research school was being built and what was later known as the “Sommerfeldschule” (Yeang, 2013, p. 46).
In the quest for a theory of radio wave propagation in Germany, it was the Göttingen-trained physicist who most contributed to it. If one accepts that the mathematical physics tradition took root at Göttingen at that time, then it is certainly not surprising that several of its physicists paved the way for theoretical and experimental studies on radio propagation and the ionosphere. Yet the brilliance of Göttingen's successes should not be allowed to obscure the fact that, at least until the mid-1920s, after Appleton's discovery of the ionosphere (or the Heaviside–Kennelly layer), there was almost no university centre for studies of radio propagation. Karl Försterling's career is especially interesting in this regard, for his decision to promote theoretical studies on the ionosphere at the University of Cologne in 1925 opened the way for theories of immense significance. Försterling, a Göttingen graduate who specialised in the theory of electromagnetic waves, asked his pupil Hans Lassen to investigate the variation in ion density in this layer (Keil, 1982). As a result, Lassen not only published several papers on the formation of an ionised layer by ultraviolet light and its effect on the radio wave propagation – in a similar way to Chapman (Lassen, 1927) – but these papers also led him to formulate, independently from Appleton and others, a general magneto-ionic theory of wave propagation (Dieminger, 1974, pp. 2085–2086; Yeang, 2013, pp. 257–259).
Like at Cambridge, Göttingen's sound training in mathematical physics helped to expand the field of geomagnetism. The conditioning nature of this relationship is clearly illustrated by the research career of a Göttingen-trained physicist, Julius Bartels (Bullen, 1981; Dieminger, 1964). It was Bartels, having been trained by the cream of the Göttingen mathematical physics crop (with professors such as Landau and Max Born), who drew on the mathematical theory of statistics while working as a professor at Eberswalde, where statistical methods for geomagnetic research were first introduced. Until its application by Bartels in around 1930, this theory was regarded as an important scientific tool, but virtually nobody had seen its potential for strengthening the rigour of geomagnetic inferences. Bartels, however, soon realised that a proper interpretation of the causes of temporal geomagnetic variations required the due application of the most modern statistical tools. Accordingly, he devised statistical procedures that permitted geomagneticians to discriminate between the geomagnetic variations caused by both wave and particle solar radiation (Bartels, 1932). Another, not less important, route by which Bartels' influence broadened and expanded the field of geomagnetism was through the application of his statistical procedures to elucidate the so-called atmospheric tides. Geomagneticians subsequently determined that these small, worldwide air pressure oscillations could be generated by both gravitational tidal forces from the Moon and the Sun and thermal forces caused by heat absorption and emission linked to solar radiation (Chapman and Lindzen, 1969). The joint publication by Bartels and Chapman titled Geomagnetism (a two-volume standard work of geophysics) in 1940 is a clear exponent of how mathematical physics expanded, rather than limited, geomagnetic horizons.
The diversity of research programmes and centres is one of the most remarkable, distinctive features of the third tradition, the laboratory-based experimental physics. In Britain alone, there were no fewer than 24 physics laboratories and 15 academic engineering laboratories, mostly electrical engineering, at the end of the 19th century (Sviedrys, 1976, pp. 416 and 431–432) – a figure that grew considerably with the development of the UK's National Physics Laboratory (NPL) and the electrical and electronic firms in the interwar years. Variety and quantity were also the predominant pattern in the United States and Germany. However, a closer reading unveils notable contrasts. While in Britain (and Germany, to a great extent) the university physics laboratories, rather than the electrical engineering ones, became the prime loci of the laboratory-based radio research, in the US efforts were focused on both electrical engineering schools and corporate and military laboratories around a single hub in the Washington, D.C., area.
4.1 Britain
In Britain, the years following World War I sparked state support for, and organisation of, radio research. The establishment of the Radio Research Board (RRB) by the British government in 1920 was motivated by the following three factors of utmost importance: first, the interest in securing long-distance communication throughout the vast British Empire; second, the urge to promote research into valves (whose manufacture considerably increased during the war); and third, the firm conviction in the potential of radio technology. From its inception, the RRB concentrated radio activities in three main centres, namely Ditton Park at Slough, Aldershot Station, and the NPL at Teddington (Gardiner et al., 1982; Pyatt, 1983, pp. 91–99). What was initially a civil-type research enterprise progressively turned into military, security-based research in the 1930s. The consequences of this steady militarisation were more conspicuous in the fields of thermionic valves, direction finding and atmospherics, upon which safety in air and sea navigation depended, rather than in radio wave propagation, which held an honourable place as research field in academia, especially at Cambridge and King's College, London (Anduaga, 2009a, pp. 18–39). The British radar programme, initiated by Robert Watson Watt and which was crucial in the course of World War II, epitomised this militarisation (Watson Watt, 1957; Pellinen and Brekke, 2011, Porter et al., 2018).
Of the several respects in which the experimental tradition differed from the mathematical physics, two are worthy of note. First, more than half of the experimentalists – among them, Edward V. Appleton, Charles T. R. Wilson, John A. Ratcliffe, and Gordon M. B. Dobson – were Natural Science Tripos graduates and had little knowledge about advanced mathematical physics, especially about electromagnetic theory, compared to their colleagues from the Mathematical Tripos. This is illustrated clearly by a rather obscure historical episode that describes Appleton's efforts to explain how it is that medium- and high-frequency radio waves could propagate beyond optical scopes. From 1928 to 1932, Appleton drew up several versions of a magneto-ionic theory containing equations for the complex refractive index and polarisation of radio waves in two propagation media, i.e. an ionised gas and a magnetic field. It is well known, as Appleton himself publicly acknowledged it, that his theory was mathematically modelled by Douglas R. Hartree in 1932 (in fact, his equations came to be known as the Appleton–Hartree equations; Appleton, 1932; Hartree, 1931); yet, it is much less known that he was also assisted by his pupil, Austrian theoretical physicist Wilhelm Altar, in 1925–1926. Appleton appropriated Altar's ideas, hiding Altar's contribution in both his papers and private correspondence. Altar, in fact, wrote a draft that included the magneto-ionic equations in the dielectric tensor form, as Gillmor (1982) has credibly shown. In 1947, Appleton was awarded the Nobel Prize for Physics for his “investigations of the physics of the upper atmosphere, especially for the discovery of the so-called Appleton layer” (Gillmor, 1982; Wilkes, 2011). At their laboratories at King's College and Cambridge, Appleton and Ratcliffe demarcated a clearly experimental territory and circumscribed practices within it, turning to skilful mathematicians to pursue the most demanding and arduous mathematical challenges.
A second defining feature of the British experimental physicists was their ability to open new avenues of research. A comparison of their practices and those of other atmospheric researchers can shed much light in this regard. Both diffraction theorists and theoretical geomagneticians tended to seek rigorous mathematical solutions to problems derived from straightforward, highly idealised physical models. Yet, the lack of verisimilitude often led them to dead ends. This was in sharp contrast to experimentalists who, in their pursuit of the coherence between physical models and experimental data, promoted new stimuli by analogy and replication. The case of Cambridge professor of physics Joseph John Thompson shows how much the mysteries of the upper atmosphere could be revealed in a laboratory setting. Around 1900, Thompson used electrical discharges to recreate physical processes in vacuum tubes and vessels at Cavendish Laboratory, University of Cambridge (Kim, 1995; Crowther, 1926, 1974). The streams of electrical particles, in theory irradiated by the Sun and impinging upon the higher atmosphere (thereby forming the aurorae), were those that supposedly appeared in the experiments with ionised gases (Lord Rayleigh, 1941, pp. 590–591). His investigations on electrical discharges, conducting gases, and cathode rays not only was the result of the signature style of the Cavendish school, characterised by an approach based on reductionism and microphysics, but also opened new perspectives to upper atmospheric geophysics (Kim, 2002). By studying wave propagation through ionised means, the new turn-of-the-century microphysics reinvigorated the Scandinavian interest in magnetism and aurorae, which, in turn, invigorated the emerging area of radio communications (Gillmor, 1997; Buchwald, 1985; Beynon, 1969).
The effects of this invigoration were almost immediate. The demand of technical training in wireless communication in Britain was such that, in the 1910s, many universities provided wireless telegraphy teaching and made some inroads on radio research as well. In order to fully appreciate the magnitude of this activation, the new research prospects in upper atmospheric geophysics have to be borne in mind. Largely as a result of William Eccles' and other engineering scientists' investigations, the range of radio research lines became broader than that of the diffraction theorists, who were more concerned with longer distances of transmission. In 1912, Eccles formulated the ionic refraction theory that explained three kinds of wireless phenomena, namely the diurnal variations in atmospherics, the wave transmission along the Earth's surface, and questions of directivity in Marconi's aerial (Eccles, 1912). As never before, and stimulated without doubt by the turn towards experimental microphysics, the new wireless investigations encompassed seemingly unrelated phenomena, such as diurnal variation in atmospherics, wave transmission, and antenna directivity. What was initially a geometric optic problem in the 1920s gradually extended to the qualitative behaviour of any kind of wireless phenomenon.
So the expansion that occurred in atmospheric physics in the 2 decades following the milestone of Marconi's first transatlantic radio transmission must be regarded, by any standards, as impressive. The interest in atmospheric electricity and the electrical field of thunderstorms which had languished in the late 19th century was revived. When Charles T.R. Wilson invented the cloud chamber to reproduce cloud formation at the Cavendish Laboratory, his concerns circled around condensation questions of ion properties, atmospheric electricity, fog, rain, hail, and so on (Longair, 2016, pp. 156–159; Harrison, 2011). His intellectual universe was an incessant circular movement between ionic questions and the nature of atmospheric phenomena. By the late 1920s, his research lines had moved on from cloud chamber and conductivity work to lightning and the polarity and charge of thunderstorms (Blackett, 1960, pp. 282–287; Halliday, 1970; Beynon, 1969). Others followed him. Thomas W. Wormell carried out experimental studies of atmospherics and analysed the effects of thunderstorms and lightning discharges on the Earth's electric field. The Cambridge laboratory, headed by Ernest Rutherford, achieved special notoriety in 1932 when John Cockcroft and Ernest Walton split the atom for the first time (Longair, 2016). At Cavendish Laboratory, the British-entrenched culture of observation was somewhat laid aside. The upper atmosphere became a realm of experimentation rather than of mere observation.
4.2 The United States
Laboratory-based experimental physics in the United States was characterised by the variety of research programmes and centres. The most detailed summary on the history of ionospheric physics in this country (Waynick, 1975, pp. 12–13) mentions four radio laboratories, two governmental and two industrial, established before 1930. The number of laboratories increased from four to 18 and as such were created in universities and military centres during and after the war. It was mainly in two centres, namely the US National Bureau of Standards (NBS) and the Naval Research Laboratory, Washington, D.C. (NRL). Both centres were governmental and military in origin, and that cluster of researchers carried out the bulk of investigations. In addition, two radio companies established important electrical engineering laboratories, i.e. the Bell Telephone Laboratories, Inc. and RCA Communications Systems.
There are several reasons why the United States proved to be a congenial site for the development of electrical engineering. Most important is the presence of committed communities of physicists and telegraph engineers who were able to mould institutions within which the speciality could grow. The first departments of electrical engineering had been established at the universities of Missouri and Cornell in the 1880s. No less important is the fact that the US Navy promoted actions to modernise its fleets as a result of post-bellum industrialisation. Some top-ranking officers soon perceived the need for incorporating wireless telegraphy into the navy. Only well after 1900, when physicists and electrical engineers discovered unanticipated industrial applications of wireless telegraphy, did the field find a secure place in the US Navy (Aitken, 1976, pp. 1–28, Aitken, 1985; Hong, 1996, 2001).
An early episode, which clearly illustrates the spirit of cooperation distinguishing the relations between the NBS and NRL and their shared interests in electrical engineering, concerns the creation of the US Naval Wireless Telegraphic Laboratory (NWTL; Austin, 1912). Plans for this laboratory had been gestating from 1899 when Marconi began to install wireless telegraph sets on warships. But it was not until 1908, when the incorporation of radio technology was recognised as a major need for effective communication, that naval authorities proposed NBS establish a laboratory at the NBS building in Washington, D.C. Military considerations helped spur the interest of NBS, but there were also technical reasons, such as personnel and facilities for electrical research, which prompted its engineers to pursue this avenue (Cochrane, 1966, p. 140). Technical evaluations, measurement of wireless devices, and equipment tests proved to be specialised tasks for men who were convinced that competence in electrical engineering was not at odds with physical experimentation. In fact, the point became explicit in Louis Austin's career. From 1908 to 1923, in which he held the post of NWTL's director, Austin conducted long-distance radio measurements and studied the effect of humidity, temperature, and magnetic storms on long-range transmissions. Coming from someone with formal qualifications in physics and laboratory experience (both gained in Europe), his interest in (or approach to) physical experimentation was a logical choice (Weinmeister, 1922, pp. 42–43). Moreover, his approach almost certainly represented the collective opinion of NBS. It is hard to imagine that a different route to experimental physics had then been taken in other NBS laboratories.
The existence of a deeply engrained local tradition was apparent at NBS. The progeny of the 19th century Office of Standard Weights and Measures, NBS had adopted Europe as a model (Cochrane, 1966, pp. 49–62). Incorporated into the Department of Commerce from 1903, its policy had been directed towards self-sufficiency and competitiveness, and that aim had been achieved through the creation of measuring instruments and experimental methods to measure electrical quantities. It was, precisely, the models of the British NPL and the German Physikalisch-Technische Reichsanstalt (Imperial Institute of Physics and Technology) that were emulated to create the NBS in 1901 as a means of increasing the international competitiveness of national industry. Innovation was an important step, and radio soon became a field of experimentation. From 1914, a split in the NBS electrical division gave way to the radio section, with the young physicist John Howard Dellinger taking charge of the laboratory. In 1920, interest concentrated on high-frequency waves, especially those used in experimental broadcasting stations, and later shifted to long-distance shortwave transmission. By 1927, NBS had launched multi-laboratory programmes to study how fading, skip-zone effect, and atmospheric disturbance influenced radio wave propagation (Snyder and Bragaw, 1986).
The cooperative nature of the relations between NBS and other agencies in the field of radio wave propagation is illustrated clearly by an episode centred on radio amateurs. It was the American Radio Relay League (ARRL), the largest association of radio hobbyists in the US, which provided the use of its transmitting stations for NBS's fading tests in 1920. At the request of NBS researchers, representatives of NBS, ARRL, NRL, and the Department of Terrestrial Magnetism (DTM) discussed the way to conduct fading tests collectively (Kruse, 1920, pp. 5–6). While ARRL was in charge of arranging shortwave stations, coordinating operations, standardising schedules, and collecting data, NBS constructed experiments and analysed all the gathered data. To this end, dozens of stations were mobilised (Yeang, 2013, pp. 115–122). As professional radio scientists learnt to devise experiments, the US amateur radio enthusiasts' role became indispensable. Paradoxically, the formation of a NBS–NRL–DTM network in Washington in the early 1920s only hastened the process, since it allowed amateur radio enthusiasts to participate in large-scale scientific experiments for the first time, and many of them who operated in the eastern US at the time seized the opportunity.
An even more important route by which the Washington network stimulated radio innovation at NBS is illustrated by a case history that begins with a skilful engineer named Theodore R. Gilliland. It was Gilliland, coming from Illinois to NBS in 1928, who turned the experiments probing the Heaviside layer into a regular ionospheric sounding programme. From 1928 to 1931, Gilliland drew upon the DTM's pulse echo method and the NRL's NFK transmitting station in Anacostia, Washington, D.C., to conduct daily pulse echo measurements (Gilliland et al., 1932, pp. 286–309). As the DTM's interest in the ionosphere faded, NBS felt it necessary to set up its own pulse transmitter (Synder and Bragaw, 1986, p. 207). Over the next few years, Gilliland improved the efficiency of measurement. An initial pulse echo device, with which one could hardly observe the continuous variation in the ionospheric virtual heights, soon evolved into one of far greater efficiency and with automatic recording. His aim was to record critical frequencies as the magneto-ionic theory considered them as an index of the layer's electron density. It is a mark of the NBS expertise that, when a new technology for distinguishing critical frequencies was needed, Gilliland thus invented the automatic sweep frequency recorder, and it became the ionospheric sounder par excellence in the 1930s–1950s (Gilliland, 1933; Jones-Imhotep, 2001).
Had US ionospheric physics depended solely on the patronage of Carnegie or on the endeavour made by NBS and radio amateurs, it might not have prospered as it did in the interwar years. Hence, if one has to explain its success, epitomised in the confluence of two traditions in the 1920s, one should look not only to private and government patronage but also to the military roots of ionospheric research. In particular, one should look into the NRL and the plans of Thomas Edison behind its foundation in 1923. NRL's genesis is usually associated with a suggestion by Edison to the secretary of the US Navy in 1915 that the navy needed new facilities and funds for research. Although the process was complex (Allison, 1979, 1981, pp. 17–38), I will very briefly summarise what historian Bruce William Hevly called the Edisonian conception of industrial research (Hevly, 1987, p. 16):
From its establishment until World War II, NRL did not operate under the assumption that pure research was an immediate necessity for military strength and the national defence. Researchers (at the NRL) did not believe they were engaged in the kind of research that went on at universities. Rather, the assumptions behind the foundation of the NRL lie in the turn of the century context that produced agricultural experiment stations, naval engineering stations, Edison's own research laboratories, and the early industrial laboratories at General Electric, Du Pont, and American Telephone and Telegraph in the US. These attitudes were supported by much of the US's experience in World War I because it meant a fierce intensification of industrial organisation and production. Industrialists and scientists were thrust into closer contact with one another (see, e.g., Wise and Whitney, 1985).
Information flowed freely between two divisions of the NRL, i.e. the Radio Division and the Heat and Light Division, which later became Physical Optics. This flow of information was much like that at the DTM, and in both places, collaboration between divisions was practised as often as circumstances allowed. Albert Hoyt Taylor, leader of the Radio Division, was captivated by the Edisonian suggestion while working at Western Electric and directing wartime naval communications (Taylor, 1948, 1960). In 1918, he was appointed the head of the Aircraft Radio Laboratory at the Naval Air Station in Anacostia. There, he recruited several amateur radio operators (such as Louis A. Gebhard and Leo C. Young), who had worked on shortwave frequencies during the war and later joined NRL (Gebhard, 1979, pp. 31–34 and 45–53). Their mission at NRL was to promote high-frequency radio for naval communications. Taylor's group was unfamiliar with academia. Amateur radio operators were so highly regarded that their knowledge counted as much as Taylor's doctoral degree and perhaps more than his bachelor's degree in electrical engineering (Hevly, 1987, p. 21).
Scientific policy in Edward O. Hulburt's Light Division was guided by the idea that ionospheric research should be technically useful and, likewise, focused on applied science. From the outset, Hulburt tried to incorporate the data collected by Taylor's group into the growing body of mathematical theory dealing with ionospheric phenomena. In time, his division became the seat of the most academic research. “None of us were engineers,” recalled Hulburt years later, “we were pure research physicists” (Hevly, 1987, p. 26). Yet the disparity of styles was never seen as synonymous with confrontation. Hulburt and Taylor even worked together on such engineering issues as the skip distance, i.e. the shortest distance permitting radio signals to travel from the transmitter to the receiver by ionospheric reflection. In 1926, their alliance culminated in a theory to determine the density of free electrons in the ionosphere (Taylor and Hulburt, 1926). By 1928, the gap was conspicuous between a division that contributed to the physical theory of the ionosphere and the Radio Division, with its massive collection of data on signal strength and transmission ranges at various frequencies. That gap widened over the next decade, at least in terms of quality and originality. The rift was due in part to the successful solving of the problem of skip distance – Hulburt and Taylor had modified Joseph Larmor and Eccles' ionic refraction theory. But it owed more, especially in the 1930s, to the formulation of Hulburt's theory that postulated the action of solar ultraviolet radiation as the causal agent for atmospheric ionisation (Hulburt, 1928, 1938). The success of his ideas is reflected in the NRL's reorientation towards basic research and innovation. Hulburt's theories provided the framework for the experimental programme with V-2 rockets that NRL promoted after World War II (Hevly, 1987).
For the present purposes, the main contribution of Hulburt's applied physics lies in its function as a model of transition between the Edisonian model of industrial research and the academic model that would emerge in the postwar years. Unlike Taylor's group or the NBS researchers, Hulburt's division was made up of holders of physics doctorates who had no affiliation to industry or magnetic observatories. Hulburt himself earned a doctoral degree in physics at Johns Hopkins University (Hevly, 1987, pp. 28–29; Hevly, 1994, pp. 144–145). After 2 years in the army, Hulburt did radio research for 6 years at Johns Hopkins University and the University of Iowa, during which he saw the importance of combining theory, testing, and development (Howard, 2012). Hulburt's approach to the study of solar terrestrial relations contrasts with the geomagnetic concerns of his British colleagues. Chapman, for example, regarded the ionosphere as the ideal setting to use his experience in magnetic observation and Cambridge mathematical physics knowledge. On the contrary, Hulburt arrived at atmospheric ionisation as part of the NRL promotion of high-frequency radio for naval communications. Nevertheless, his contribution to the mathematical physics theory of the ionosphere can by no means be overlooked (Hulburt, 1974). The institutional realities each had to overcome were certainly very different.
Although the Washington network earned its reputation in ionospheric physics chiefly for research bureaus, there were other important institutions in which wave propagation studies were promoted, in varying degrees, through several common interests. I refer to the industrial laboratories of the Bell Telephone Company (founded in 1877), Western Electric (WE, 1872), the American Telephone and Telegraph (AT&T, 1885), and the Radio Corporation of America (RCA, 1919). As a rule, the emphasis, in these corporate initiatives, is on exclusively commercial development, with the optimisation of the conditions of wave transmission as the main objective. It is a mistake to underestimate these laboratories as minor agents in the elucidation of the ionospheric layers. In fact, industrial laboratories were typical in the radio and electrical engineering departments, who shared interests and issues with university and military laboratories (McMahon, 1984, pp. 133–174).
The importance of the Washington setting as a propelling force in the history of ionospheric physics in the US must be stressed again. The involvement of the federal government in the physical sciences and the opening of research careers related to terrestrial physics in the capital were trends to which physicists could not close their eyes, even if their prime interest was elsewhere (Reingold, 1991; Hevly, 1994, p. 144). It also seems that, among the young newcomers who aspired to posts of professional research in Washington, data collection and recording were more appealing than doing research at universities. This was, in part, an inevitable consequence of the US's 19th century westward expansion, which had led the government to gather physical data and to establish research bureaus for those and other ends in the Washington area (Dupree, 1986, pp. 289–299). Undoubtedly, the same is true of the approaches of Edison and other industrialists; many engineers of industrial laboratories (such as Bell Telephone, General Electric, or AT&T) must take much credit for the style of experimental investigation that their successors successfully implemented. The effect of all this is indisputable. Studies of radio wave propagation were fostered beneficially, favouring their confluence with the Humboldtian tradition of terrestrial physics in the 1920s.
4.3 Germany
The excellence of laboratory-based radio science in the age of the Second German Reich has almost never been doubted. It was an age in which at least three groups of physicists and electrical engineers, led by Adolf Slaby, at the Technical University of Berlin, and Ferdinand Braun and Jonathan Zenneck, at the University of Strasbourg, all for mainly academic reasons, and Adolf Koepsel and Carl Rode, in industrial settings, at the instigation of the military, conducted experimental work on wireless telegraphy. It was an age of an intensive search for means to generate electromagnetic waves through at least four basic technologies, namely spark transmitters, arc transmitters, high-frequency alternators, and vacuum tube transmitters. It was also an age in which not only a dense network of technical universities and several wireless companies but also the German postal, telephone and telegraph administration (Reichspostministerium), operating maritime radio services through the high-power transmitter in Norddeich, were all promoters of experimental studies. Among the wireless companies were Siemens and Halske, Germany's largest manufacturer of radio technology, through research groups such as those of Koepsel and (later) Walter Schottky working on electronic tube noises, the “Allgemeine Elektricitäts-Gesellschaft” (AEG), the “Gesellschaft fur drahtlose Telegraphie”, better known as Telefunken, which intensively worked for the German navy, and its subsidiary TRANSRADIO SenderSysteme Berlin AG, focused on shortwave overseas transmission (Friedewald, 2000; Thumm, 2006).
From the start, the threat of what was perceived as a worldwide and perilous monopoly of the Marconi Company was clear for Germany. Despite the Germans' innovative talent in wireless telegraphy, successive German governments strove to rectify what the regarded as a disproportional accumulation of Britain's technological power, which undermined their interests of a policy of imperial expansion and communication. The arguments adduced by the government and industry to establish Telefunken in 1903 were an attempt to counteract British hegemony (Rüger, 2007; Anduaga, 2009). In Germany, two bodies were especially engaged in this mission for varied reasons. The first was the navy, which believed that wireless was more appropriate for ships than land stations, and that its implementation would help to circumvent a hypothetical communications blockade by the British forces in a world war scenario. Aside from naval interests, the government valued the potential of wireless as a tool to communicate with its colonies in East Asia and South-West Africa (Namibia) without depending on Britain's worldwide telegraph network. Germany sought to escape the British net by devising their own wireless systems and by laying their own submarine cables (Hobsbawm, 1987; Pickworth, 1993; Klein-Arendt, 1996).
As far as ionospheric studies are concerned, alliance rather separation between industry and academia characterised Germany's experimental physics tradition. To all intents and purposes, Zenneck's career at the chair of experimental physics at the Technical University of Munich (from 1913 until 1936) became the pacesetter. The scholarly reputation of Zenneck and his group have a special significance for our understanding of German ionospheric physics in the interwar years. From a theoretical viewpoint, Zenneck's better-known accomplishment was the diffraction theory of radio wave propagation, to which Zenneck contributed before Sommerfeld, proposing that ground resistance played a crucial role in propagation (Zenneck, 1907). Not less important was his role in the first echo soundings in Germany carried out by his group (led by Georg Goubau in 1929) and their measurements of echo height and critical frequency, magnetic polarisation of radio waves, and radio wave propagation forecasting, among other subjects (Dieminger, 1974, pp. 2087–2089). However, for the purposes of this paper, it is perhaps more significant to see the rise of Zenneck and his group as one aspect of the role of industry and the navy as sources of influence and patronage – from 1895 to 1905, he assisted Braun at Strasbourg, conducting wireless experiments to establish a maritime radio service in Cuxhaven, and from 1907 to 1911, he conducted industrial research at the Badische Anilin- und Sodafabrik (BASF). Then, as a captain of the marines, he and Braun were sent to the US as technical advisors in a patent case involving Telefunken – all experiences that provided access to the most up-to-date wireless technology (Dieminger, 1961; Schlegel, 2014).
The new style of experimental radio science was most evident in Zenneck's group, whose members tended to weave close relationships with industry. Although payment in the German technical universities was not determined by the weight of these links, industrial disciplinarity and the access to leading-edge technologies and operational resources seem to have provided the physicists with an important incentive to conduct radio and ionospheric research in universities. This is the case of Hans Rukop, a doctoral student of Richard Mie at Greifswald, who joined Zenneck at Munich in 1913 to work on arc generators and high-frequency machines (Blumtritt, 2005). Industrial immersion was a constant trait in Rukop's career, and as early as 1914 he began working for Telefunken, where he was able to establish a high-vacuum laboratory. Such expectations created in an industrial setting had continuity during the war, when he opened several small factories to produce vacuum tubes, and in the 1920s, when he became head of all Telefunken laboratories. It is true that such accomplishments appear to be unrelated to his appointment in 1927 as professor at the new Institute for Technical Physics at the University of Cologne, but his subsequent achievements in shortwave transmission, radar, and the analysis of echoes from ionospheric reflection cannot obscure the fact that the new institute was built thanks to the collaboration of Telefunken (Rukop, 1928).
It was clearly in its industrial connections that the strengths of Zenneck's group, as a centre of radio ionospheric research, lay. For their investigations, Zenneck drew on the experimental transmitter of the Herzogstand station in the Bavarian Alps, which was installed and operated by C. Lorenz AG, an electrical firm that specialised in radio and vacuum tube manufacture. A primary competitor of Telefunken, the firm developed a radio navigation system (known as the Lorenz beam) which opened wide prospects of progress for aircraft radio business (Klawitter, 2002, pp. 85–96). A whole group of Zenneck's pupils based their postgraduate work on observations from Herzogstand. In addition to Goubau and his automatic recorder, it is worth noting that Johannes Plendl, who first worked as a radio engineer for Telefunken, developed the Lorenz beam landing system and made radio wave propagation forecasts for the German air force (Trenkle, 1991). It is also known that the head of this radio wave propagation unit was another of Zenneck's pupils, Walter Dieminger, the future director of the Central Counselling Office for Radio Communication, which was to monitor the ionosphere until the end of World War II. However, the most eminent of this group would be Karl Rawer, then a young doctoral student, who formulated a theory on the reflection of vertical incident radio waves and who worked so deftly with Plendl to optimise shortwave communications in the early 1940s that the French Navy invited him to establish the Service de Prévision Ionosphérique (Ionosphere Prediction Service) in Germany's French zone after the war (Reinisch, 2014). Although most of their research papers were discussed only in academic circles, there is much evidence that industry and the military stimulated ionospheric investigations and oriented their character (Dieminger, 1974, 1975).
The diversification of ionospheric research in Germany would not have been so notable had it not become so widespread just at a time, in the mid- and late 1920s, when radio engineering groups and centres were beginning to flourish. And how ironic this was, for these accomplishments owed much to Göttingen-trained physicists. For example, in addition to the cases mentioned above, no one did more to promote radio wave propagation and ionospheric investigations in Germany than Karl Willy Wagner, whose doctorate at Göttingen enabled him to pursue a brilliant career as professor at the Technical University of Berlin. It was through establishing the Heinrich Hertz Institute for Oscillation Research in 1928 that Wagner provided a favourable institutional setting in Berlin to conduct research in the fields of mechanical, electromagnetic, and acoustic oscillations. It was also through this institute that Wagner organised an expedition to Trömso in Norway during the International Polar Year (1932/1933; Schlegel and Lühr, 2014). As ionospheric research became increasingly integrated in upper atmospheric physics, Wagner's group maintained a lively interest in the influence of auroral disturbances on radio propagation conditions. While other sections engaged in high-frequency engineering, acoustics, and mechanics, this group formed the core of the new Department of Radio Propagation of the German Post Office (Reichspostministerium), under the direction of Friedrich Vilbig (Dieminger, 1974, p. 2090). The transition from academia to the civil world became a hallmark of German ionospheric research. While radio engineering largely flourished in industrial and military centres in the United States, technical universities set the pace in Germany.
In radio science, renown was more readily gained in technical universities, with their acknowledged scholarly journals, than in industries, where results of experimental tests and observations, when they could be conducted at all, were published in obscure and, often, company journals that few read. This does not mean, however, that they fell short of the level of academic research results. Perhaps the most notable example is electrical engineer Hans Ernst Mögel, who worked for TRANSRADIO at the transcontinental wireless station in Beelitz. Mögel was able to compare shortwave reception disturbances with geomagnetic data in a statistical way. As a result, he explained the geomagnetic influence on shortwave communication and introduced the concept of “short-time disturbances” in an article published in 1930 in a domestic journal (Telefunken Zeitung and in the German language) that, 5 years later, saw US engineer John Howard Dellinger publish similar results with no mention of Mögel's achievements; this omission notwithstanding, the phenomenon is known as Mögel–Dellinger Effect (Mögel, 1930; Traxler and Schlegel, 2014). By comparison with Zenneck's research subjects, which he regarded as “ionospheric physics”, Mögel's approach to research was applied. Mögel's duties at TRANSRADIO included ensuring reliable operations, investigating disturbances in shortwave transmissions, determining optimal transmission frequencies, and shortwave propagation forecasting.
Mathematical physicists and experimentalists were only a part, albeit a fundamental one, of the attainment of the confluence. Opportunely for Germany, Britain, and the US, there was a last tradition – an observatory-based data recording tradition – that substantially contributed to the advancement of terrestrial physics knowledge, at least from the mid-19th century. Then, Alexander von Humboldt's calls for global observations were attended by Carl Friedrich Gauss and Wilhelm Weber's venture, termed the Magnetischer Verein, in which over 20 observatories from all around the world conducted simultaneous observations of the Earth's magnetic field, with Göttingen as the hub (Wolfschmidt, 2005; Jungnickel and McCormmach, 1986, Vol. 1, pp. 63–77). With the foundation of the British Association for the Advancement of Science, this large-scale science approach received a great deal of support in Britain, resulting in the organisation of Edward Sabine's movement, known as the magnetic crusade, and John Herschel's programme of simultaneous meteorological observations. All these initiatives embodied a Humboldtian style of observation and analysis of scientific variables being suitable for observatory sciences (Cawood, 1979; Morrell and Thackray, 1981, pp. 353–70).
5.1 Britain
In Britain, the observatories of Greenwich, Kew, Lerwick, and Eskdalemuir show the scope and scale of this tradition. Greenwich, the seat of observational astronomy from its foundation, had a modest magnetic section which was transferred to Abinger in 1925. Kew, the centre of geomagnetic training, was geared towards the calibration and verification of instruments, as well as the systematic recording of magnetic fields and electrical potential gradient (Simpson, 1928; Barrell, 1969, p. 171). Yet, emphasis on investigation at Kew was by no means insignificant as compared to its main competitors, i.e. Greenwich and the Scandinavian observatories. Kew director Charles Chree earned his colleagues' respect not only for his prolificacy (over 80 magnetic papers) but also for combating speculation and conjectures, so profuse in geomagnetic science, with a zeal and meticulousness quite unknown at that time. Eskdalemuir and Lerwick benefited from the fact that electric currents associated with magnetic disturbances reached the greatest concentration at those latitudes in the Shetland Isles (Blackwell, 1958; Crichton, 1950). Their records of atmospheric electricity, one of the most esteemed observation series in Britain, and the study of aurorae, ozone, and meteorology, all reflected the commitment to a non-experimental, data recording practice (Harrison, 2003, p. 13).
With the appreciable legacy of Britain's terrestrial magnetism at their disposal and the magnetic records increasing in substantial amounts, the benefits of the observational tradition are evident. The reliable observations of potential gradient at Kew became the largest data bank of its kind in the world (Chree, 1915). Fortunately for Britain, the observatories recruited some of Cambridge's best young brains as assistants. Yet, if the methodological influence of observatories seems clear, the way by which it is exerted is far from it. How far did these observatories enable, say, Chapman to determine the causes of lunar and solar variations of terrestrial magnetism – the basis of his dynamo theory – in 1919? Or to what extent did the systematisation of magnetic and electric observations conduct scientists in the 1920s to formulate theories which, in accuracy and depth, questioned the estimates made until then?
This fact is unquestionable: the value of such an influence rested not so much on its incentive role for radio research as on the accumulation of geomagnetic data. This does not necessarily imply that observatories underestimated the potential of radio as a tool for observation; indeed, Lerwick was one of the three stations endowed with equipment for direction-finding and atmospherics (Harper, 1950, p. 313). But, in the case of Lerwick, the core of the research programme was implemented by the Department of Scientific and Industrial Research (DSIR) instead of the Meteorological Office, on which observatories normally depended. Meteorologists and geomagneticians' readiness to engage in radio research was by no means apparent. When George Clark Simpson, a world authority on atmospheric electricity, took charge of the Meteorological Office in 1920, he separated weather service from radio research. At this point, radio research and meteorology in all respects parted company in Britain.
Ironically, it was in these very years when a division was completed, that the relationship between geomagnetism and radio physics became closer than ever. Behind this lay, in part, the fact that theoretical geomagneticians pursued a global view of atmospheric physics. The early career of the most prominent geomagnetician, Sydney Chapman, illustrates the reach of this pursuit well. Chapman worked as an assistant at Greenwich from 1910 to 1914. The position was rewarding, both financially and with regards to status (Cowling, 1971, pp. 62–63; Anduaga, 2009b). Yet, at that time, at the age of only 22, he soon began to doubt whether he would concentrate on astronomy or on pure mathematics. But his role in the modernisation of the magnetic section and his contacts with Professor Arthur Schuster proved instrumental in defining his research career. Chapman drew on data from over 20 observatories to publish his first results on solar and lunar magnetic variations in terms of dynamo theory and, thereby, extend Schuster's theory to the lunar case. He systematised the preceding theories of Stewart and Schuster and tested them against the collected data, a tedious task entailing the classification of observations. Chapman's strong commitment with research is in sharp contrast with the detachment of most of his colleagues at Greenwich: “It seems an unfortunate fact”, he stated, “that the efforts of magneticians are unduly devoted to the accumulation of data, the time and labour spent in their discussion being proportionally inconsiderable” (Chapman, 1919, p. 2). Being, no doubt, fully conscious of these shortcomings, Chapman accepted the challenge, and his theories always rested firmly on data interpretation. His stay at Greenwich and his repeated use of worldwide data helped to cement his belief in the observational-type tradition (Vestine, 1967, p. 22).
5.2 The United States
The style of the observatory-based data recording tradition was most evident at the Carnegie Institution of Washington (CIW), where requests for the large-scale geomagnetic campaigns by Humboldt and Sabine were taken into serious consideration (Good, 1994b). Although financing was not promoted by the US government as it was in many European centres at that time, self-esteem and the need for complete and coordinated surveys seem to have provided geoscientists with sufficient incentive to attract great philanthropists such as the steel baron Andrew Carnegie. A survey of the entire Earth's magnetic field was coveted information, and the CIW emerged as an appropriate agency for coordinating international research in 1902.
Such magnetic surveys were encouraged by Louis A. Bauer in countries like Canada and China and European colonies in Africa. Born to German parents who migrated to Ohio, Cincinnati, Bauer sought to instill the CIW with a theoretical and observational profile. This dual profile fitted in with Bauer's background. Bauer went to Berlin to study physics and geophysics under Max Planck and Wilhelm von Bezold. His doctoral thesis was a mathematical analysis of the geomagnetic secular variation (Fleming, 1932). After graduating in 1895, he returned to the US and took a position as the first chief of the Coast and Geodetic Survey's Division of Terrestrial Magnetism (DTM). In this position, Bauer established five magnetic observatories. The CIW profited from his experience. It is true that his primary goal was to trace “the intimate relationship between terrestrial magnetism and other sciences, such as meteorology, geology, and astronomy” (Bauer, 1904, pp. 203–204). But his continuing effectiveness in organising magnetic expeditions (130 between 1905 and 1921) and editing a leading journal (Terrestrial Magnetism, later the Terrestrial Magnetism and Atmospheric Electricity, and now the Journal of Geophysical Research) cannot obscure the fact that the CIW had confined its foci of interests to geomagnetism and geochemistry by the end of World War I.
Of the various institutions which engaged in this observatory-based recording strategy, the most significant was the DTM (Reingold, 1979; Good, 1994a). From its foundation to World War I, the DTM largely concentrated on the terrestrial magnetism programme, especially on world magnetic survey. So, in many respects, continuity rather than change characterised the pre-war period (or Bauer's stage). But in some less obvious ways the decade of 1918–1928 marked a turning point. It coincided with the appointment of the CIW's new president, John C. Merriam, and the DTM's new director, John Adam Fleming. Now the DTM's explicit objectives were much ambitious – to combine observation work and laboratory investigations, to encourage basic research for industrial practice, and to concentrate on the Earth's atmosphere and crust. In all these respects, it was successful. The recruitment of young physicists with backgrounds in radio science and engineering combined with important funds for equipments stimulated the atmospheric experimental research that eclipsed such earlier practices as endorsed by geomagneticians and geologists. A very promising project for cooperation between DTM, radio amateurs, and universities was materialised with the pulsed radio sounding of the ionosphere. And, from 1926 on, an inter-institutional programme enabled the regular radio sounding of the upper atmosphere.
Although the enthusiasm for field experimentation was paramount, it cannot be understood in isolation from the hidden bonds and personal links that characterised the Washington network (Hevly, 1994; Gillmor, 1994, p. 141). Most of the DTM's key players were associated with Johns Hopkins University and the University of Minnesota. Almost all of them had belonged to, or cooperated with, the NBS and the NRL, whose radio activities had assumed new vigour from the end of the war. William F. G. Swann, for example, taught at Minnesota from 1918 to 1923, where he devised pulse echo experiments. There he met student Merle Antony Tuve and assistant professor Gregory Breit, both of whom were much impressed with Swann's method. Breit, for his part, studied at Johns Hopkins University with Edward O. Hulburt, and then arranged for Tuve to complete his thesis at Johns Hopkins University, after which he brought him to into DTM (Hull, 1998, pp. 32–35). Lloyd Berkner studied electrical engineering at the University of Minnesota, worked at NBS, and joined DTM with Tuve's support (Hales, 1992, pp. 4–6). This sustained assistance can be interpreted as a way of reinforcing established institutional fabrics, but it also produced lasting benefits for ionospheric physics and the radio industry (Tuve, 1974). Like Cambridge and Göttingen in Europe, Johns Hopkins University and the University of Minnesota were the two high-level technical centres that acted as drivers for ionospheric physics advances in the United States.
5.3 Germany
When Gauss, then a 55 year-old professor at Göttingen, turned his interest to terrestrial physics, he introduced (along with Weber) absolute units of magnetic measurements for the first time, overcoming the limitations of earlier pre-calibrated magnetic instruments. In founding a magnetic section at the Göttingen observatory, he was making an operation of distinctive astuteness, which complements the magnetic work of his predecessor Tobias Mayer. That he was correct in laying the foundations of the German geomagnetic tradition in the 1830s is apparent in the formation of the Magnetischer Verein, which promoted measurements of the Earth's magnetic field in many parts of the world (Schröder and Wiederkehr, 2002, pp. 445–447). But the appropriateness of Gauss and Weber's initiatives cannot be better illustrated than by the ability of the Magnetischer Verein to collect measurements from 57 observatories (18 of which were from outside Europe) from 1836 to 1841, which constitutes the first major international collaboration in the field of geophysics. When, in 1897, the geomagnetic department was separated from the observatory, the idea was to transform Gauss and Weber's plan into a geomagnetic institute. From then on, proficiency in terrestrial physics came to be measured by proficiency in field research and observation rather than at the lectern.
However, from 1898, when Emil Wiechert was appointed director of the newly created Institut für Geophysik (Institute of Geophysics), little geomagnetic research was performed at Göttingen (Siebert, 1997). Wiechert, coming from Königsberg where he had addressed the question of an Earth core and the mass distribution in the Earth's interior, focused his efforts on seismic studies, with a special emphasis on the construction of automatically registering seismographs. In this field, Wiechert's institute soon reached international recognition (Wiechert, 1906). Geomagnetic sciences did not regain strength at the institute until the 1930s, with the decisive fact there being the appointment of Bartels as director, and he applied statistical mathematics to geomagnetic and meteorological series. From that point the Institut für Geophysik (Institute of Geophysics) recovered Gauss's essence, as Bartels himself admitted, by applying the Gaussian-frequency distribution and other statistical tools to the study of diurnal magnetic variations (Bartels, 1932).
The activities of several Prussian institutes in Potsdam provide further evidence of the extent to which German scientists cultivated the terrestrial physics tradition. There is conclusive evidence that the Meteorological Institute sponsored a magnetic survey of northern Germany. Of course, solar–terrestrial connections and physics of the high atmosphere had by no means been strangers in the 19th century observatories (Voppel, 1974; Schröder and Wiederkehr, 2002). At the Potsdam Magnetic Observatory, concepts such as its director Max Eschenhagen's elementary waves had been much discussed, especially his idea that they were the result of electric currents in the upper atmosphere (Eschenhagen, 1897). During the next decades, however, these questions gathered renewed interest at Potsdam; it was his successor, Adolf Schmidt, for example, who analysed the geomagnetic effects of a phenomenon (the ionosphere's tides) that, although well known at the time, required statistics and mathematical methods in its treatment. In fact, it was as clear to Schmidt as it had been to the leading geomagneticians of 19th century Prussia that observatories should approach science in the way that Humboldt approached his notion of a global Earth – by seeking the solar–atmospheric–terrestrial connections (Bartels, 1946). Moreover, it is remarkable that, at a time when the dependence of geophysical research on specialised institutes grew rapidly in Germany, so many scholars and assistants from Potsdam institutes maintained close links with the University of Berlin. Schmidt's professorship of geophysics, offered by an invitation from Berlin, was perhaps the most prominent. In any event, it seems that the observatory–university connection nourished the vision of a global physics in the geosciences.
By World War II, Germany's terrestrial physics tradition (Humboldtian in its roots, though arguably also Gaussian) had followed, to all effects, a fecund path. It had stimulated international networks and work of outstanding quality. Its theories, most notably Gauss' general theory of terrestrial magnetism and Bartels' treatise on geomagnetism (Chapman and Bartels, 1940), were taught as basic principles in geophysical courses, and its accomplishments were the fruit not only of scholarship but also research. Yet, although such accomplishments may have appeared successful at that time, its main achievement seems to be the contribution to the confluence between geomagnetism and radio science with regards to ionospheric physics.
In this article, I have examined the way in which an emerging, physical research speciality, ionospheric physics, flourished in Britain, Germany, and the United States in the 4 decades before World War II. I have intended to show that interwar achievements in ionospheric research in those countries and their disparate styles cannot be explained satisfactorily in terms of either the discovery of layers or the formulation of theories. Of course, the finding, first by Appleton and Barnett in England and then by Tuve and Breit in the United States, of the ionospheric layers in the mid-1920s was a historical watershed, and it is tempting to add that the subsequent magneto-ionic theories of radio wave propagation were resulting milestones. But why did such accomplishments take place at that precise time and in those places? Although this paper's aim is not to explain the causes of individual achievements, it does seek to analyse success of collective, institutional, and national scientific endeavours. By placing emphasis on patterns, styles, and relations, rather than on facts, feats, and events, this paper aims to show just how much of the transmission of practices and ideas from one generation to another fell into oblivion. After all, explanations of the supposed birth of ionospheric physics after 1925 that invoke discovery narratives are very likely to be weak constructs.
Given that the role of scientific traditions has not received sufficient attention from historians of the geosciences, I have discussed, in some detail, the intellectual and social threads of continuity which led geoscientists to open research areas in the way they did. I have suggested that a dynamic conception of scientific tradition provides new vistas for the study of geosciences history. There was no sudden occupation or discovery of ignored or desert areas; there were innovative research groups and/or schools within deep-rooted scientific traditions. The idea of an emerging speciality, springing up from a historical discovery or a great theory, is inadequate. There was, instead, a confluent movement – a transformation of important research areas from within. In retrospect, it was the extraordinary ability of Britain, Germany, and the United States to fuse theory and experimentation and to combine radio science and geomagnetic studies within and outside academia by considering and strengthening the interests of their domestic radio industries and the militaries.
In attempting to describe this confluent movement, I have paid special attention to the reconceptualisation of objects of research within certain scientific traditions. This movement can be interpreted as the confluence of four rooted traditions – Cambridge school's mathematical physics, Göttingen's mathematical physics, laboratory-based experimental physics and Humboldtian-style terrestrial physics – in which investigators transformed, from within, research areas related to geomagnetism and radio wave propagation.
In our time, the history of emerging specialties is often expressed in explanatory terms and in which individual achievements have an instrumental but causal role. The emphasis on individuals and dispersed collectivities rather than geographically located research groups and schools arises, in part, from sociologists' search for explanations in their idea that “social and conceptual innovations are uniformly intertwined” (Geison, 1981, pp. 31–32). In this paper, by adopting categories like the scientific tradition, it is important to bear in mind that such concepts cannot serve, to paraphrase Secord (1986, p. 261), as final arbiters of explanation; a scientific tradition, such as those described above, is in essence a descriptive category for tracking threads of intellectual and social continuity among patterns of changing relations. Although it cannot explain those patterns in causal terms, it does suggest effective ways of exploring these threads.
No data sets were used in this article.
The author declares that there is no conflict of interest.
I wish to thank the editor, Kristian Schlegel, for his assistance and the reviewers, Norbert Jakowski, Gregory A. Good and one anonymous referee, for their helpful and constructive suggestions.
This paper was edited by Kristian Schlegel and reviewed by Norbert Jakowski, Gregory A. Good, and one anonymous referee.
Aitken, H. G. J.: Syntony and Spark: The Origins of Radio, Wiley, New York, 1976.
Aitken, H. G. J.: The Continuous Wave: Technology and American Radio, 1900–1932, Princeton University Press, Princeton, New Jersey, 1985.
Allison, D. K.: The origin of the Naval Research Laboratory, US Naval Inst. Proc., 105, 119–139, 1979.
Allison, D. K.: New Eye for the Navy: The Origin of Radar at the Naval Research Laboratory, National Research Laboratory, Washington, D.C., 1981.
Anduaga, A.: Wireless and Empire. Geopolitics, Radio Industry, and Ionosphere in the British Empire, 1918–1939, Oxford University Press, Oxford, 2009a.
Anduaga, A.: Sydney Chapman on the layering of the atmosphere: conceptual unity and the modelling of the ionosphere, Ann. Sci., 66, 333–344, 2009b.
Anduaga, A.: Geophysics, Realism, and Industry. How Commercial Interests Shaped Geophysical Conceptions, 1900–1960, Oxford University Press, Oxford, 2016.
Appleton, E. V.: Wireless studies of the ionosphere, P. I. Electr. Eng., 71, 642–650, 1932.
Austin, L. W.: The work of the U.S. Naval Radio-Telegraphic Laboratory, J. Am. Soc. Naval Eng., 24, 122–141, 1912.
Barrell, H.: Kew Observatory and the National Physical Laboratory, Meteorol. Mag., 98, 171–180, 1969.
Bartels, J.: Statistical methods for research on diurnal variations, Terr. Mag. Atm. Elec., 37, 291–302, 1932.
Bartels, J.: Adolf Schmidt, 1860–1944, J. Geophys. Res., 51, 439–447, 1946.
Bauer, L. A.: Proposed International Magnetic Bureau, CIW Year Book [for] 1903, 2, 203–212, 1904.
Beynon, W. J. G.: The Physics of the Ionosphere, Sci. Prog., 57, 415–433, 1969.
Blackett, P. M. S.: Charles Thomson Rees Wilson, 1869–1959, Bio. Mem. Fell. Royal Soc., 6, 269–295, 1960.
Blackwell, M. J.: Eskdalemuir Observatory: The first fifty years. Meteorol. Mag., 87, 129–132, 1958.
Blumtritt, O.: Rukop, Hans, in: Neue Deutsche Biographie, Duncker & Humblot, Berlin, 2005.
Buchwald, J.: From Maxwell to Microphysics: Aspects of Electromagnetic Theory in the Last Quarter of the Nineteenth Century, University of Chicago Press, Chicago, 1985.
Bullen, K. E.: Bartels, Julius, in: Dictionary of Scientific Biography, edited by: Gillispie, C. C., Charles Scribner's Sons, New York, 1981.
Cawood, J.: The magnetic crusade: Science and politics in early Victorian Britain, Isis, 70, 493–518, 1979.
Chapman, S.: Solar and lunar diurnal variations of terrestrial magnetism, Philos. T. R. Soc. S-A, 218, 1–118, 1919.
Chapman, S. and Bartels, J.: Geomagnetism, Oxford University Press, Oxford, 1940.
Chapman, S. and Lindzen, R. S.: Atmospheric Tides: Thermal and Gravitational Springer, Dordrecht, 1969.
Chree, C.: Atmospheric electricity potential gradient at Kew Observatory, 1898 to 1912, Philos. T. R. Soc. S-A, 215, 133–159, 1915.
Cochrane, R. C.: Measures for progress. A history of the National Bureau of Standards, National Bureau of Standards, Washington, D.C., 1966.
Cowling, T. G.: Sydney Chapman, 1888–1970, Biogr. Mem. Fell. Royal Soc., 17, 53–89, 1971.
Crichton, J.: Eskdalemuir Observatory, Meteorol. Mag., 79, 337–340, 1950.
Crowther, J. G.: Research work in the Cavendish Laboratory in 1900–1918, Nature, 118, 58–60, 1926.
Crowther, J. G.: The Cavendish Laboratory, 1874–1974, MacMillan Press, London, 1974.
Dieminger, W.: Jonathan Zenneck, R. Oldenbourg, München, 1961.
Dieminger, W.: Julius Bartels, Naturwissenschaften, 51, 229–230, 1964.
Dieminger, W.: Early ionospheric research in Germany, J. Atmos. Terr. Phys., 36, 2085–2093, 1974.
Dieminger, W.: Trends in early ionospheric research in Germany, Philos. T. R. Soc. S-A, 280, 27–34, 1975.
Dupree, A. H.: Science in the Federal Government: A History of Policy and Activities to 1940, Johns Hopkins, Baltimore, 1986.
Eccles, W. H.: On the diurnal variations of the electric waves occurring in nature, and on the propagation of electric waves round the bend of the Earth, P. R. Soc. London, 87, 79–99, 1912.
Eckert, M. and Märker, K.: Arnold Sommerfeld, Wissenschaftlicher Briefwechsel, Band 1: 1892–1918, Deutsches Museum, Munich, 2000.
Eschenhagen, M.: Uber schnelle periodische Veränderungen des Erdmagnetismus von sehr kleiner Amplitude, Sitzber. K. Preuss. Aka, 32, 678–686, 1897.
Fleming, J. A.: Louis Agricola Bauer (1865–1932), Science, 75, 452–454, 1932.
Forman, P. and Hermann, A.: Arnold Sommerfeld, in: Dictionary of Scientific Biography, edited by: Gillispie, C. C., Charles Scribner's Sons, New York, 12, 525–532, 1975.
Friedewald, M.: The beginnings of radio communication in Germany, 1897–1918, J. Radio St., 7, 441–463, 2000.
Gardiner, G. W., Lane, J. A., and Rishbeth, H.: Radio and space research at Slough, 1920–1981, J. I. Electron, Radio Eng., 52, 111–121, 1982.
Gebhard, L. A.: Evolution of Naval Radio-Electronics and Contributions of the Naval Research Laboratories, Naval Research Laboratory, Washington, D.C., 1979.
Geison, G. L.: Scientific change, emerging specialties, and research schools, Hist. Sci., 19, 20–40, 1981.
Gilliland, T. R.: Note on a multi-frequency automatic recorder of ionosphere heights, J. Res. Nat. Bur. Stand. 11, 561–566, 1933.
Gilliland, T. R., Kenrick, G. W., and Norton, K. A.: Investigations of Kennelly-Heaviside layer heights for frequencies between 1600 and 8650 kilocycles per second'. P. Ire., 20, 286–309, 1932.
Gillmor, C. S.: The place of the geophysical sciences in nineteenth century natural philosophy, EOS T. Am. Geophys. Un., 56, 4–7, 1975.
Gillmor, C. S.: Wilhelm Altar, Edward Appleton, and the magneto-ionic theory, P. Am. Philos. Soc., 126, 395–423, 1982.
Gillmor, C. S.: Federal Funding and Knowledge Growth in Ionospheric Physics, 1945–81, Soc. Stud. Sci., 16, 105–133, 1986.
Gillmor, C. S.: The big story: Tuve, Breit, and ionospheric sounding, 1923-1928, in: The Earth, the Heavens and the Carnegie Institution of Washington, edited by: Good, G. A., The American Geophysical Union, Washington D.C., 133–141, 1994.
Gillmor, C. S.: The formation and early evolution of studies of the magnetosphere, in: Discovery of the Magnetosphere, edited by: Gillmor, C. S. and Spreiter, J. R., The American Geophysical Union, Washington, D.C., 1–12, 1997.
Good, G. A.: The breadth, height, and depth of the geosciences and space sciences at the Carnegie Institution of Washington, in: The Earth, the Heavens and the Carnegie Institution of Washington, edited by: Good, G. A., American Geophysical Union, Washington, D.C., xi–xiii, 1994a
Good, G. A.: Vision of a global physics: The Carnegie Institution and the first World Magnetic Survey, in: The Earth, the Heavens and the Carnegie Institution of Washington, edited by: Good, G. A., American Geophysical Union, Washington, D.C., 29–36, 1994b.
Good, G. A.: The assembly of geophysics: Scientific disciplines as frameworks of consensus, Stud. Hist. Philos. Sci., 31, 259–292, 2000.
Green, A. L.: Early history of the ionosphere, A.W.A. Tech. Review, 7, 177–228, 1946.
Hales, A. L.: Keith Edward Bullen, 1906–1976, Rec. Australian Ac. Sci., 4, 42–64, 1979.
Halliday, E. C.: Some memories of Prof. C. T. R. Wilson, English pioneer in work on thunderstorms and lightning, B. Am. Meteorol. Soc., 51, 1133–1135, 1970.
Harper, W. G.: Lerwick Observatory, Meteorol. Mag., 79, 309–314, 1950.
Harrison, G.: The cloud chamber and CTR Wilson's legacy to atmospheric science, Weather, 66, 276–279, 2011.
Harrison, R. G.: Twentieth-century atmospheric electrical measurements at the Observatories of Kew, Eskdalemuir and Lerwick, Weather, 58, 11–19, 2003.
Hartree, D. R.: The propagation of electromagnetic waves in a refractive medium in a magnetic field, P. Camb. Philos. Soc., 27, 143–162, 1931.
Hevly, B. W.: Basic Research within a Military Context: The Naval Research Laboratory and the Foundations of Extreme Ultraviolet and X-Ray Astronomy, 1923-1960, Ph. D. thesis, Johns Hopkins University, Baltimore, 1987.
Hevly, B. W.: Building a Washington network for atmospheric research, in: The Earth, the Heavens and the Carnegie Institution of Washington, edited by Good, G.A., American Geophysical Union, Washington, D.C., 143–148, 1994.
Hobsbawm, E. J.: The Age of Empire, 1875–1914, Pantheon Books, New York, 1987.
Hong, S.: Styles and credit in early radio engineering: Fleming and Marconi on the first transatlantic wireless telegraphy, Ann. Sci., 53, 431–465, 1996.
Hong, S.: Wireless: from Marconi's Black-Box to the Audion, The M.I.T. Press, Cambridge, Mass., 2001.
Howard, J. N.: Edward O. Hulburt: Frederick Ives Medalist, 1955, Opt. Photonics News, May, 22–23, 2012.
Hulburt, E. O.: Ionization of the upper atmosphere of the Earth, Phys. Rev., 31, 1018–1037, 1928.
Hulburt, E. O.: Photoelectric ionization in the ionosphere, Phys. Rev., 53, 344–351, 1938.
Hulburt, E. O.: Early theory of the ionosphere, J. Atmos. Terr. Phys., 36, 2137–2140, 1974.
Hull, M.: Gregory Breit, 1899–1981, Nat. Acad. Sci. Bio. Mem., 74, 27–56, 1998.
Jones-Imhotep, E. C.: Communicating the Nation: Northern Radio, National Identity and the Ionospheric Laboratory in Cold War Canada, PhD thesis, Harvard University, Cambridge, Massachusetts, 2001.
Jungnickel, C. and McCormmach, R.: Intellectual Mastery of Nature: Theoretical Physics from Ohm to Einstein, vols. 1–2, University of Chicago Press, Chicago, 1990.
Kargon, R. H.: Science in Victorian Manchester. Enterprise and Expertise, Johns Hopkins University Press, London, 1977.
Keil, K.: Lassen, Hans, in: Neue Deutsche Biographie, edited by: Historischen Kommission bei der Bayerischen Akademie der Wissenschaft, Duncker & Humblot, Berlin, 1982.
Kenrick, G. W. and Pickard, G. W.: Summary of progress in the study of radio wave propagation phenomena, P. Ire., 18, 649–668, 1930.
Kim, D. W.: J. J. Thomson and the emergence of the Cavendish School, 1885–1900, Brit. J. Hist. Sci., 28, 191–226, 1995.
Kim, D. W.: Leadership and Creativity. A History of the Cavendish Laboratory, 1871–1919, Kluwer Academic, Dordrecht and London, 2002.
Klawitter, G.: 100 Jahre Funktechnik in Deutschland. Wiss.-und Technik-Verl, Berlin, 2002.
Klein, F.: Plan eines physikalisch-technischen Instituts an der Universität Göttingen, Z. Ver. Dtsch. Ing., 40, 102–105, 1896.
Klein-Arendt, R.: Kamina ruft Nauen! Die Funkstellen in den deutschen Kolonien 1904–1918, W. Herbst, Köln, 1996.
Kruse, S.: The Bureau of Standards-ARRL tests of short wave radio signal fading, QST, 4, 5–37, 1920.
Kushner, D.: Sir George Darwin and a British School of Geophysics, Osiris, 8, 196–223, 1993.
Lassen, H.: Die taglichen Schwankungen des Ionisationszustandes der Heaviside-Schicht, Elektrische Nachrichten-Technik, 4, 174–179, 1927.
Lightman, B. V.: The Dictionary of Nineteenth-Century British Scientists, Thoemmes Continuum, London, 2004.
Longair, M. S.: Maxwell's Enduring Legacy: A Scientific History of the Cavendish Laboratory, Cambridge University Press, Cambridge, 2016.
Love, A. E. H.: The transmission of electric waves over the surface of the Earth, Philos. T. R. Soc. Lond., 215, 105–131, 1915.
Macdonald, H. M.: The bending of electric waves round a conducting obstacle, P. R. Soc. Lond., 71, 251–258, 1903.
McMahon, A. M.: The Making of a Profession: A Century of Electrical Engineering in America, The Institute of Electrical and Electronics Engineers Press, New York, 1984.
Mimno, H. R.: The physics of the ionosphere, Rev. Mod. Phys., 9, 1–44, 1937.
Mögel, H.: Uber die Beziehungen zwischen Empfangsstorungen bei Kurzwellen und den Storungen des magnetischen Feldes der Erde, Telefunken-Zeitung, 11, 14–31, 1930.
Morrell, J. and Thackray, A.: Gentlemen of Science: Early Years of the British Association for the Advancement of Science, Clarendon Press, Oxford, 1981.
Neuenschwander, E. and Burmann, H. W.: Die Entwicklung der Mathematik an der Universität Göttingen, in: Die Geschichte der Verfassung und der Fachbereiche der Georg-August-Universität zu Göttingen, edited by: Schlotter, H. G., Vandenhoeck & Rupprecht, Göttingen, 141–159, 1994.
Nicholson, J. W.: On the bending of electric waves round the Earth, Philos. Mag., 19, 276–278, 1910.
Olesko, K. M.: Physics as a Calling: Discipline and Practice in the Königsberg Seminar for Physics, Cornell University Press, Ithaca, N.Y., 1991.
Pellinen, R. and Brekke, A.: Introduction `The History of Ionospheric Radars', Hist. Geo Space. Sci., 2, 113–114, https://doi.org/10.5194/hgss-2-113-2011, 2011.
Pestre, D.: Studies of the ionosphere and forecasts for radiocommunications. Physicists and engineers, the military and national laboratories in France (and Germany) after 1945, Hist. Technol., 13, 183–205, 1997.
Pickworth, G.: Germany's Imperial Wireless System, Electron. World Wirel., 99, 427–432, 1993.
Porter, J. G., De Bruyn, W., and Saltzman, E. S.: Eddy flux measurements of sulfur dioxide deposition to the sea surface, Atmos. Chem. Phys., 18, 15291–15305, https://doi.org/10.5194/acp-18-15291-2018, 2018.
Pyatt, E. C.: The National Physical Laboratory: A History, Hilger, Bristol, 1983.
Rayleigh, Lord John William Strutt: Joseph John Thomson, Obituary Not. Fell. R. Soc., 3, 587–609, 1941.
Reingold, N.: National science policy in a private foundation: the Carnegie Institution of Washington, in: The Organization of Knowledge in Modern America, 1860-1920, edited by: Oleson, A. and Voss, J., Johns Hopkins University Press, Baltimore, 313–341, 1979.
Reingold, N.: Science, American Style, Rutgers University Press, New Brunswick, 1991.
Reinisch, B. W.: Karl Rawer: space research and international cooperation – Laudation on the occasion of the 100th birthday of Professor Karl Rawer, Adv. Radio Sci., 12, 221–223, https://doi.org/10.5194/ars-12-221-2014, 2014.
Rüger, J.: The Great Naval Game: Britain and Germany in the Age of Empire, Cambridge University Press, Cambridge and New York, 2007.
Rukop, H.: Die Telefunkenröhren und ihre Geschichte, in: 25 Jahre Telefunken; Festschrift der Telefunken-Gesellschaft, 1903-1928, edited by: Telefunken G.m.b.H., [Berlin], 114–154, 1928.
Schlegel, K.: Ionospheric research in Germany prior to Karl Rawer, Adv. Radio Sci., 12, 225–230, https://doi.org/10.5194/ars-12-225-2014, 2014.
Schlegel, K. and Lühr, H.: Willy Stoffregen – An early pioneer of advanced ionospheric and auroral research, Hist. Geo Space. Sci., 5, 149–154, https://doi.org/10.5194/hgss-5-149-2014, 2014.
Schröder, W. and Wiederkehr, K. H.: Geomagnetic research in the 19th century, Acta Geod. Geophys. (Hungary), 37, 445–466, 2002.
Secord, J. A.: The Geological Survey of Great Britain as a research school, 1839-1855, Hist. Sci., 24, 223–265, 1986.
Siebert, M.: Geschichte des Instituts fiir Geophysik in Gottingen, in: Zur Geschichte der Geophysik in Deutschland Jubiläumsschrift zur 75jährigen Wiederkehr der Gründung der Deutschen Geophysikalischen Gesellschaft, edited by: Borngën, M., Junge, A., Schweitzer, J., and Neunhöfer, H., Deutsche Geophysikalische Gesellschaft (DGG), Hannover, 101–114, 1997.
Simpson, G. C.: Charles Chree, 1860–1928, P. R. Soc. London, 122, vii–xiv, 1928.
Simpson, G. C.: Sir Arthur Schuster, 1851–1934, Obituary Not. Fell. R. Soc. Lond., 1, 409–423, 1935.
Snyder, W. F. and Bragaw, C. L.: Achievement in Radio: Seventy Years of Radio Science, Technology, Standards, and Measurement at the National Bureau of Standards, National Bureau of Standards, Boulder, Colo., 1986.
Sommerfeld, A.: Uber die Ausbreitung der Wellen in der drahtlosen Telegraphie, Ann. Phys-Berlin, 28, 665–736, 1909.
Sommerfeld, A.: Gesammelte Schriften, Bd. 4, F. Vieweg, Braunschweig, 1968.
Sviedrys, R.: The rise of physics laboratories in Britain, Hist. Stud. Phys. Biol., 7, 405–436, 1976.
Taylor, A. H.: Radio Reminiscences: A Half Century, U.S. Naval Research Laboratory, Washington, D.C., 1948, reprinted in 1960.
Taylor, A. H. and Hulburt, E. O.: The propagation of radio waves over the Earth, Phys. Rev., 27, 189–215, 1926.
Thumm, M.: Historical German contributions to physics and applications of electromagnetic oscillations and waves, in: History of Wireless, edited by: Sarkar, T. K., Mailloux, R. J., Oliner, A. A., Salazar-Palma, M., and Sengupta, D. L., John Wiley & Sons, Hoboken, N. J., 327–348, 2006.
Traxler, F. and K. Schlegel: Hans Mögel, Transradio, and the Mögel Dellinger Effect, Radio Sci. Bull., 351, 53–57, 2014.
Trenkle, F.: Zum 90. Geburtstag von Hans Plendl, Funkgeschichte, 78, 3–5, 1991.
Tuska, C. D.: Historical notes on the determination of distance by timed radio waves, J. Franklin Inst., 237, 1–20, 83–102, 1944.
Tuve, M. A.: Early days of pulse radio at the Carnegie Institution, J. Atmos. Terr. Phys., 36, 2079–2083, 1974.
Vestine, E. H.: Geomagnetism and solar physics, in: Sydney Chapman, Eighty: from his Friends, University of Colorado, Boulder, 19–23, 1967.
Voppel, D.: Hundert Jahre Erdmagnetischer Dienst in Norddeutschland, in: Zur Geschichte der Geophysik: Festschrift zur 50jährigen Wiederkehr der Gründung der Deutschen Geophysikalischen Gesellschaft, edited by: Birett, H., Heibig, K., Kirtz, W., and Schmuker, U., Springer Verlag, Berlin, 139–148, 1974.
Warwick, A.: Masters of Theory: Cambridge and the Rise of Mathematical Physics, The Chicago University Press, Chicago, 2003.
Watson, G. N.: The diffraction of electric waves by the Earth, P. R. Soc. London, 95, 83–99, 1918–1919a.
Watson, G. N.: The transmission of electric waves round the Earth, P. R. Soc. London, 95, 546–563, 1918–1919b.
Watson Watt, R. A.: Three Steps to Victory: A Personal Account by Radar's Greatest Pioneer, Odhams Press, London, 1957.
Waynick, A. H.: The early history of ionospheric investigations in the United States, Philos. T. Roy. Soc. A, 280, 11–25, 1975.
Weinmeister, P. F. W.: Poggendorffs Biographisch-Literarisches Handworterbuch 5, Verlag Chemie, Berlin, 1922.
Whittaker, E. T.: Macdonald, Hector Munro, Obituary Not. Fell. R. Soc., 11, 551–558, 1935.
Whittaker, J. M.: George Neville Watson, Biogr. Mem. Fell. Royal Soc., 12, 521–530, 1966.
Wiechert, E.: Das Institut für Geophysik der Universität Göttingen, in: Die Physikalischen Institute der Universität Göttingen. Festschrift im Anschlusse an die Einweihung der Neubauten am 9. dezember 1905, edited by: Akademia Georgia Augusta, B. G. Teubner, Leipzig and Berlin, 119–188, 1906.
Wilkes, M. V.: Sir Edward Appleton and Early Ionosphere Research, Notes Rec. Roy. Soc., 2, 113–114, 2011.
Wilson, D. B.: Experimentalists among the mathematicians: Physics in the Cambridge Natural Sciences Tripos, 1851-1900, Hist. Stud. Phys. Biol., 12, 325–371, 1982.
Wise, G. and Whitney, W. R.: General Electric, and the Origins of US Industrial Research, Columbia Press, New York, 1985.
Wolfschmidt, G.: Vom Magnetismus zur Elektrodynamik: herausgegeben anlässlich des 200. Geburtstags von Wilhelm Weber (1804-1891) und des 150. Todestages von Carl Friedrich Gauss (1777-1855), Schwerpunkt Geschichte der Naturwissenschaften, Mathematik und Technik, Hamburg, 2005.
Yeang, C. P.: The study of long-distance radio-wave propagation, 1900–1919, Hist. Stud. Phys. Biol., 33, 369–404, 2003.
Yeang, C. P.: Probing the Sky with Radio Waves: From Wireless Technology to the Development of Atmospheric Science, University of Chicago Press, Chicago, 2013.
Zenneck, J.: Über die Fortpflanzung ebener elektromagnetischer Wellen längs einer ebenen Leiterfläche und ihre Beziehung zur drahtlosen Telegraphie, Ann. Phys-Berlin, 23, 846–866, 1907.