the Creative Commons Attribution 4.0 License.
the Creative Commons Attribution 4.0 License.
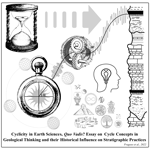
Cyclicity in Earth sciences, quo vadis? Essay on cycle concepts in geological thinking and their historical influence on stratigraphic practices
Daniel Galvão Carnier Fragoso
Matheus Kuchenbecker
Antonio Jorge Campos Magalhães
Claiton Marlon Dos Santos Scherer
Guilherme Pederneiras Raja Gabaglia
André Strasser
The archetype of a cycle has played an essential role in explaining observations of nature over thousands of years. At present, this perception significantly influences the worldview of modern societies, including several areas of science. In the Earth sciences, the concept of cyclicity offers simple analytical solutions in the face of complex events and their respective products, in both time and space. Current stratigraphic research integrates several methods to identify repetitive patterns in the stratigraphic record and to interpret oscillatory geological processes. This essay proposes a historical review of the cyclic conceptions from the earliest phases in the Earth sciences to their subsequent evolution into current stratigraphic principles and practices, contributing to identifying opportunities in integrating methodologies and developing future research mainly associated with quantitative approaches.
- Article
(24264 KB) - Full-text XML
- BibTeX
- EndNote
The word “cycle” derives from the Greek term “κψκλoσ”, used to describe any circular body as well as any circular and perpetual movement of successive events or phenomena, which keep returning to their original position positions during their dynamics. Ancient Greeks described repetitive patterns to characterize the organization of almost all known processes. This idea of uniformity and continuity has been influenced by empirical observations of natural phenomena like day and night, changes in the moon phase, and seasons (Nelson, 1980).
From a historical perspective, the cycle archetype is found in several ancient traditions, attested, for instance, in lithic monuments such as Stonehenge, built-in alignment with solar and lunar cycles (Hawkins, 1963), and religious practice regulated by the seasons and their astronomical markers (Boutsikas and Ruggles, 2011). Nowadays, the cyclicity conception is widespread in many areas of knowledge. The Italian philosopher Giambattista Vico (1668–1744), for example, introduced in the 18th century the cyclic idea of history (Vaughan, 1972). According to Vico, societies develop in a similar and repetitive pattern, followed by phases of social and political organizations, going from insurrection to inevitable decline. An exciting aspect of Vico's vision is that the perpetual motion of history does not reproduce a perfectly circular pattern but a spiral: in each new cycle that begins, there is a remnant of the cycle that has ended. In the present century, Puetz (2009) proposed the “Unified Cycle Theory”, which seeks to demonstrate how cycles dominate the universe's structure, influencing various aspects of life on Earth. Through the predictability aspect of the cyclical approach, this author pursues definition of future turning points for humanity.
Explanations of sound, tone, and harmonics were among the first elements of modern physical science. This early success in description and prediction of periodic astronomical events together with an understanding of periodicity related to vibration in the production of sounds led scientists to seek periodicities elsewhere in the natural world. Today the list is extensive for phenomena in which cycles have been studied. It includes sunspot activity, tides and ocean waves, Earth tides, music, human speech, tree-ring growth, animal population changes, brain waves, heart rhythm, chemical bonding forces, climatic activity, economic growth, light and other electromagnetic wave phenomena, and geological events (Preston and Henderson, 1964, p. 415).
Cycles, rhythms, oscillations, pulsations, repetitions, or periods are examples of terms frequently used in the geological literature that reflect a profound influence of the conception of cyclicity in the Earth sciences. Whether through a historical heritage or from the various discoveries made over time, examples are plentiful to demonstrate that the idea of cycles is used to describe geological processes and products containing some characteristic repetitive patterns in the geological record.
Considering the overuse of cyclicity concepts, Dott (1992) described them as a “powerful opiate” for geologists. The criticism of the cyclic approach is that there is an innate psychological appeal to simplicity provided by rhythmically repetitive patterns that attempt to order randomness (e.g. Nagel, 1961; Zeller, 1964). It is a fact that, in many cases, cycle concepts are vaguely used in the geological literature without the commitment to defining order and periodicity. This is the case of the rock cycle, a postulate that states that the rock record itself is a product of a fundamental cycle in which igneous, sedimentary, and metamorphic rocks are continuously turned into one another (e.g. Gregor, 1992). It is understandable that, in cases like this, the concept of cyclicity is used as a device to didactically explain various complex and repetitive processes that occur on the planet (e.g. Peloggia, 2018). However, the current understanding of the processes that integrate the Earth system theorizes the existence of periodical processes, at different timescales, that emanate from the astronomical forces that make our planet interact with neighbouring celestial bodies (e.g. Hinnov, 2018) and from the complex dynamics of the Earth's interior (e.g. Mitchell et al., 2019). In this way, the occurrence of “true cycles”, which correspond to an orderly, repetitive progression of events that is unlikely to occur by chance, is increasingly being demonstrated. Many of these cycles leave a recognizable mark in the geological record, and their understanding is invaluable in the study of stratigraphic organization.
Nature vibrates with rhythms, climatic and dystrophic, those finding stratigraphic expression ranging in period from the rapid oscillation of surface waters, recorded in ripple-marks, to those long-deferred stirrings of the deep imprisoned titans which have divided earth history into periods and eras. The flight of time is measured by the weaving of composite rhythms – day and night, calm and storm, summer and winter, birth and death – such as these are sensed in the brief life of man. But the career of the Earth recedes into a remoteness against which these lesser cycles are as unavailing for the measurement of that abyss of time as would be for human history the beating of an insect's wing. We must seek out, then, the nature of those longer rhythms whose very existence was unknown until man by the light of science sought to understand the Earth [...] Sedimentation is controlled by them, and the stratigraphic series constitutes a record, written on tablets of stone, of these lesser and greater waves which have pulsed through geologic time (Barrell, 1917, p. 746).
Henceforward, cycle concepts have been essential in promoting geological knowledge and constitute one of the pillars of stratigraphy (e.g. Schwarzacher, 2000). Current stratigraphic research integrates several systematic methods to identify and interpret repetitive units of the sedimentary record (e.g. sequence stratigraphy and cyclostratigraphy). In this context, comprehension of the origin and evolution of the cyclicity concepts in stratigraphy is quite relevant and opportune. The following synthesis reviews the main works that use these concepts to interpret geological processes and their imprint in the stratigraphic record. It goes from a historical review to the current state of the art in stratigraphic principles and practices.
Studies of cyclicity in geological processes commonly seek to find periodicities in data series and explain them in terms of known natural phenomena (Preston and Henderson, 1964). The current demonstration of recurring global processes, with regular periodicity, illustrates the search for “longer rhythms whose very existence was unknown until man by the light of science sought to understand the Earth” (Barrell, 1917, p. 746). In a recent investigation into the recurrence and synchronicity of global geological events, Rampino et al. (2021) determined the existence of an Earth pulsation. The authors analysed 89 significant and well-dated geological events over the past 260 million years, including marine and non-marine biological extinctions, major oceanic anoxic events, flood-basalt eruptions, sea-level fluctuations, pulses of intraplate magmatism, and times of changes in seafloor-spreading rates and plate reorganization. Moving-window analysis evidences the presence of 10 peaks or clusters in the number of events (Fig. 1a). Between these peaks, the number of events approaches zero. Fourier analysis shows that the highest peak occurs at 27.5 Myr (99 % confidence), with a secondary signal at 8.9 Myr (Fig. 1b). Similar cycles have been determined in other studies analysing climate change (e.g. Shaviv et al., 2014), sea-level oscillations (e.g. Boulila et al., 2018), extinctions (e.g. Clube and Napier, 1996), and Earth's tectonic behaviour (e.g. Müller and Dutkiewicz, 2018). The common finding of several authors is that these cyclical events are global, correlative, and tightly coupled. According to Rampino et al. (2021, p. 6), the correlation and cyclicity of these episodes point to an essentially periodic and coordinated geological record, whose origin “may be entirely a function of global internal Earth dynamics affecting global tectonics and climate, but similar cycles in the Earth's orbit in the Solar System and in the Galaxy might be pacing these events”.
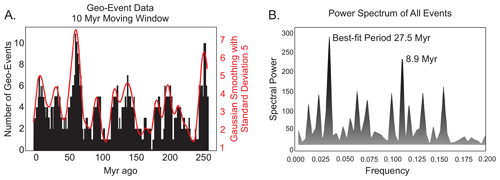
Figure 1(a) Analysis of the ages of 89 geologic events using a 10 Myr moving window centred every 0.5 Myr, with the number of occurrences that fall within the moving window computed at 1 Myr intervals. Ten clusters (peaks) are visible. In red is the Gaussian smoothing with a standard deviation of 5 Myr centred at every 0.1 Myr, with 10 peaks. (b) Fourier transform results show the highest peak in 27.5 Myr, and a strong secondary period occurs at 8.9 Myr (modified from Rampino et al., 2021).
2.1 The astronomical clock
Periodicity is one of the fundamental phenomena recorded by observant man. Cycles associated with astronomical events were among the first natural phenomena described with sufficient precision and generality that such events could be predicted for the future. Even for primitive societies, one measure of their level of scientific understanding is the accuracy of their calendars (Preston and Henderson, 1964, p. 415).
The roots of the geologists' appeal for the periodicity of natural processes may be found in the Aristotelian worldview, which expanded the human experiences of the cyclic phenomena, such as day and night, tides, and seasons (Dott, 1992). In one of the first essays about the history of geology, the classic book Principles of Geology by Charles Lyell (1797–1875) mentions this possible relationship.
When we consider the acquaintance displayed by Aristotle, in his various works, with the destroying and renovating powers of nature, the introductory and concluding passages of the twelfth chapter of his “Meteorics” are certainly very remarkable. In the first sentence, he says, “the distribution of land and sea in particular regions does not endure throughout all time, but it becomes sea in those parts where it was land, and again it becomes land where it was sea; and there is a reason for thinking that these changes take place according to a certain system, and within a certain period.” The concluding observation is as follows: “As time never fails, and the Universe is eternal, neither the Tanais, nor the Nile, can have flowed forever. The places where they rise were once dry, and there is a limit to their operations, but there is none to time. So also of all other rivers; they spring up, and they perish; and the sea also continually deserts some lands and invades others. The same tracts, therefore, of the Earth are not, some always sea, and others always continents, but everything changes in the course of time.” It seems, then, that the Greeks [...] deduced, from their own observations, the theory of periodical revolutions in the inorganic world (Lyell, 1835, pp. 21–22).
Lyell (1835) discusses the intellectual advance of ancient civilizations, such as the Hindus and the Egyptians, and highlights mainly Greek philosophy that considered the course of events on the planet to be continually repeated in perpetual vicissitude, mainly influenced by the knowledge of astronomy. The various Greek contributions to scientific knowledge reflect a strong sense of observation of astronomical cycles. Among the many examples, the studies of celestial phenomena and their potential for temporal calibrations stand out. Hipparchus of Nicaea (190–120 BC), considered by many to be the greatest of Greek astronomers, used mathematical bases to determine the length of the year and the recurrence of eclipses with relatively high precision. Credit must be given to his conclusions about the motion of the stars, which Nicolaus Copernicus (1473–1543) later attributed to the “precession of the equinoxes” (Hockey et al., 2007). Twenty centuries later, these concepts would guide the research on orbital cyclicity used to construct paleoclimatic, cyclostratigraphic, and astrochronological models (e.g. Hinnov, 2018).
2.1.1 The beginning of glacial theories
The discovery of glacial cycles is among the greatest ever made in the Earth sciences. In 1837, Louis Agassiz (1807–1873), then president of the Swiss Society of Natural Sciences, presented ideas that shocked his peers (Imbrie and Imbrie, 1979). Agassiz (1840) argued that large fragments of rock, which occurred erratically in the region of the Jura mountains, far from their areas of origin, were evidence of an ancient ice age. Although these ideas were not necessarily original, having been put forward in the 18th century by James Hutton (1729–1797) and Bernard Friederich Kuhn (1762–1825), Agassiz brought “the glacial theory of scientific obscurity to the public eye” (Imbrie and Imbrie, 1979, p. 21).
Although the conception of an ice age was fundamentally as being catastrophic, its development took place on fertile ground for ideas of the cyclical nature of geological processes. Before Agassiz's work, one of the pioneers was Jens Esmark (1762–1839). Esmark (1824) showed that massive glaciers covered different parts of Europe, sculpting the landscape, and proposed the eccentricity of the Earth's orbit as a hypothesis that caused climate change. Influenced by William Whiston's (1667–1752) contributions about the elliptical orbit, which would periodically place Earth far from the Sun, Esmark combined these findings into a consistent theory (Hestmark, 2017). The dissemination of such ideas fostered the scientific debate that continues to the present day. Research into the relationship between recurrent glaciations and orbital cycles advanced significantly with the contributions of Joseph Alphonse Adhémar (1797–1862) and James Croll (1821–1890).
Adhémar (1842) sought to explain glaciations by reinforcing the hypothesis of orbital controls, especially the precession of the equinoxes. In his book Les Révolutions de la Mer, Déluges Périodiques, he argues that the glacial periods alternated between the hemispheres, with two glaciations – one to the north and one to the south – every 23 kyr. Anticipating what is now known as thermohaline circulation, he introduced the effects of large-scale ocean currents, which link the planet's South Pole and North Pole, to explain the phenomenon of melting ice (Berger, 2012).
James Croll's works stood out for defending the astronomical theory of glacial periods based on rigorous mathematical reasoning, significantly influenced by the astronomer Urbain Leverrier (1811–1877) and his research on orbital cyclicity. Croll sought to demonstrate that precession variation, modulated by eccentricity, drastically affects the intensity of radiation received by the Earth during each season of the year (Imbrie and Imbrie, 1979). Thus, he defended the origin of glaciations based on this seasonal effect. Furthermore, Croll considered the possibility of atmospheric amplification of orbital cycles through albedo effects as the snow caps grow and of amplifying orbital effects through ocean circulation (Paillard, 2001). In 1875, in the book Climate and Time, Croll updated his theory considering the variations in the inclination of the Earth's axis (obliquity cycle). Unfortunately, without further information on the timing of these variations, his study could not provide definitive answers (Imbrie and Imbrie, 1979).
In the mid-19th century, the effects of glacial cycles were also studied, mainly on sea-level fluctuations. MacLaren (1842), for example, influenced mainly by the work of Agassiz, suggested that melting and reconstruction of the ice sheets that covered continents during glaciation should cause significant variations in the volume of the ocean. He estimated that these variations would reach magnitudes of 100 to 200 m, closely anticipating the current understanding of glacioeustasy (e.g. Sames et al., 2020). Jamieson (1865) proposed another glacial mechanism for the relative change in sea level. From his investigations in Scotland, he suggested that the weight of the ice caps must have depressed part of the crust during the glaciation, which would return to its original position during the thaw (isostatic rebound).
2.1.2 Milankovitch and the definitive return of astronomical climate models
The legacy of Croll's work served as a foundation for the Serbian Milutin Milankovitch (1879–1958). Milankovitch is one of the most well-known pioneers of planetary climatology, especially for finding a mathematical solution to correlate orbitally controlled insolation with the ice ages (Milankovitch, 1941; Paillard, 2001; Fig. 2).
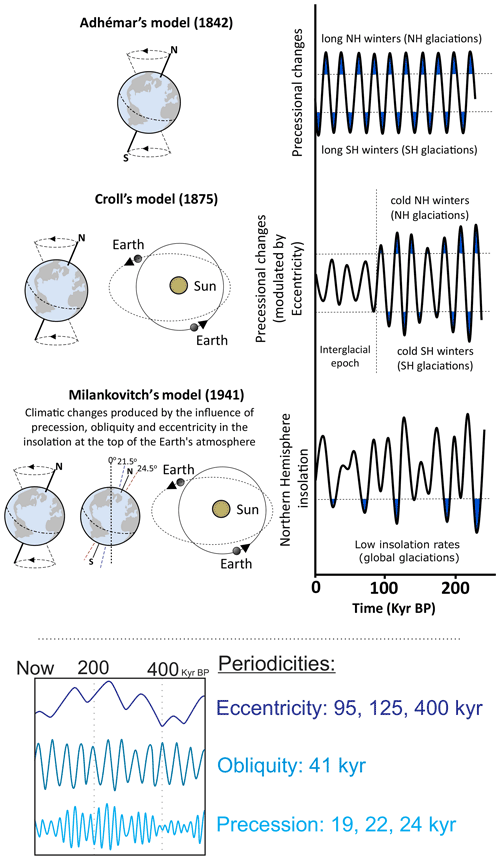
Figure 2Orbital models for glacial cycles. Adhémar's model considers only precession to explain cyclic glaciations alternating between hemispheres. Croll's model considers the interferences of eccentricity. The last is Milankovitch's model, a pioneer in determining the insolation calculated from all orbital parameters (modified from Paillard, 2001).
Milankovitch (1941) calculated the glacial–interglacial climatic oscillations as a function of solar radiation incident at the top of the atmosphere (insolation) for the last 600 kyr. While his predecessors used only eccentricity and precession, Milankovitch also included obliquity in his calculations. The triumph of Milankovitch's work was the precision, which could be tested with geological data for validation. The variations in solar radiation produce changes between colder (lower insolation rates) and warmer global climatic periods (higher insolation rates), which then influence atmospheric, hydrological, oceanographic, biological, and sedimentological processes on the Earth's surface.
Some geologists accepted that the curves proposed by Milankovitch fit the geological record. However, many others disagreed, discrediting astronomical research, remaining skeptical until studies of deep-sea cores and isotopic research started (Imbrie and Imbrie, 1979). According to the Milankovitch model, Emiliani (1955, 1966, 1978) determined that ocean temperatures fluctuated, based on a record of oxygen isotope ratios in calcitic fossils. Later, Shackleton (1967) improved the interpretation of variations in oxygen isotope ratios, suggesting that they reflect oscillations in the total volume of ice sheets during glacial cycles. Nowadays, Milankovitch's work is an essential element of deductive analysis and has become the keystone of cyclostratigraphy and astrochronology (e.g. Strasser et al., 2006). Astronomical solutions are calculated with ever-higher precision for the deep geological past (e.g. Berger et al., 1989; Laskar et al., 2011; Hinnov, 2018), and Milankovitch cycles are used to improve the geological timescale continually (e.g. Gradstein et al., 2021).
2.1.3 Astronomical forcings on the Earth system
Many astronomical cycles leave a recognizable imprint in the geological record (e.g. House, 1995, Fig. 3), ranging from twice-daily (such as tides; e.g. Kvale, 2006) to hundreds of millions of years (such as the vertical oscillation of the solar system across the galactic plane and its association with impact episodes and mass extinction events on Earth; e.g. Randall and Reece, 2014). The geochronological value of these astronomical cycles has been recognized by many authors, which has led to the rise of astrochronology (Hinnov, 2018). Astronomical dating helps reconstruct the global climate history (e.g. Westerhold et al., 2020) and is now a significant element of the geological timescale (e.g. Walker et al., 2013; Gradstein et al., 2021).
In addition to the build-up and melting of ice on the polar caps during icehouse conditions, astronomical cycles in the Milankovitch frequency band also force global processes during greenhouse times (e.g. Schulz and Schäfer-Neth, 1998; Boulila et al., 2018; Strasser, 2018; Wagreich et al., 2021). Geological records in different parts of the world suggest a strong correlation between orbital cycles and global sea-level fluctuations. The eustasy associated with astronomical forcing on Earth's climate (Fig. 4a) includes the exchange of water between the ocean and terrestrial stores, either in the form of ice (glacioeustasy; Fig. 4a) or underground and surface reservoirs (aquifereustasy and limnoeustasy; Fig. 4b), and also thermally induced volume changes in the oceans (thermoeustasy; Fig. 4c). During icehouse conditions, glacioeustasy predominates with high-amplitude sea-level fluctuations, while in a greenhouse world amplitudes are minor (e.g. Wilson, 1998; Séranne, 1999; Sames et al., 2016; Fig. 5).
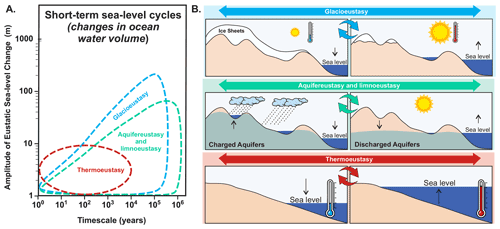
Figure 4(a) Log-scale diagram of the timing and amplitudes of the main mechanisms that control “short-term” sea-level variations. The values represented must be considered averages (modified from Sames et al., 2016); (b) schematic diagrams representing the processes that promote changes in sea level (glacioeustasy, aquifer eustasy + limnoeustasy, and thermoeustasy) during climate changes induced by orbital cycles.
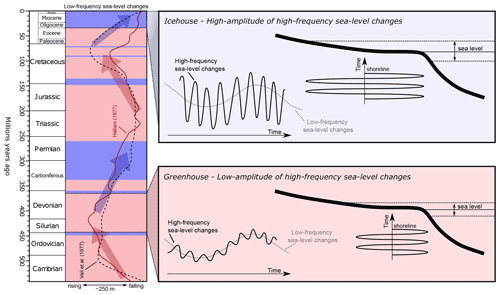
Figure 5Changing frequencies and amplitudes of eustasy. Sea-level curves, according to Vail et al. (1977) and Hallam (1977). In icehouse periods (in blue), these cycles have a high amplitude, mainly due to the effects of glacioeustasy. Eustatic oscillations have lower amplitude in greenhouse periods (in light red) since there is no significant glacial effect (modified from Wilson, 1998; Séranne, 1999; Montañez et al., 2011).
2.2 The internal gears of geodynamics
In the 18th century, during the Scottish Enlightenment, James Hutton (1726–1797) described the geological record observed in the landscape as a product of the continuous alternation of uplift, erosion, and depositional processes. The emergence of geology as an individualized science is currently linked to James Hutton's Theory of the Earth, which described the Earth as a body that acts cyclically over geological time (Chorley et al., 2009).
This uniformitarian conception has a cyclical approach, which considers a priori that geological processes present repetitive patterns (O'Hara, 2018). The most significant contributor to the spread of uniformitarian thinking, Charles Lyell, presented a fascinating tale of the Earth's internal oscillating processes. He visited the Macellum of Pozzuoli (also known as Serapis Temple – Fig. 6a) in the Italian region of Campania several times, highlighting this Roman ruin in an illustration on the frontispiece of the Principles of Geology (Fig. 6b). In the middle portion of the three remaining marble pillars, there are borings left by marine Lithophaga bivalves. According to Lyell, it is “unequivocal evidence that the relative level of land and sea has changed twice at Puzuolli, since the Cristian era, and each movement both of elevation and subsidence has exceeded twenty feet” (Lyell, 1835, p. 312). This variation of relative sea level identified by Lyell is now understood as a product of bradyseism, which corresponds to vertical ground movements (Fig. 6c) caused by successive filling and emptying of magmatic chambers in volcanic areas (Parascandola, 1947; Bellucci et al., 2006; Lima et al., 2009; Cannatelli et al., 2020).
The search for processes in the Earth's internal dynamics, and their relationship with sea-level variations, continued for many years after Hutton and Lyell. However, such research focused on finding diastrophic rhythms at large temporal and spatial scales, as Barrell (1917) mentioned: “those long-deferred stirrings of the deep imprisoned titans which have divided earth history into periods and eras”.
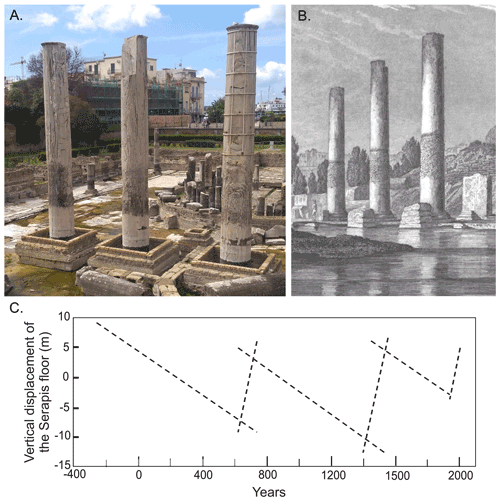
Figure 6Roman ruins of the Serapis Temple (Macellum of Pozzuoli), in Pozzuoli, Italy. (a) Recent picture. (b) The illustration on the frontispiece of volume I of Principles of Geology (Lyell, 1835). Both highlight the rough texture of the intermediate portion of the columns where bivalve wear is evident, indicating marine transgression after the temple's construction. (c) Vertical movements of the Serapis Temple show an alternating pattern of elevation and subsidence produced by bradyseism (modified from Bellucci et al., 2006).
2.2.1 Diastrophic theories and the birth of eustasy
The 18th and 19th centuries were the most scientifically active for the nascent discipline of geology. During this period, Earth's contraction was the leading theory for the origin and evolution of its morphology, such as mountain ranges. According to this conception, the Earth's radius diminished with time due to internal cooling, causing the crust to wrinkle. The theory of the Earth's cooling and contraction has been developed and modernized throughout history, with collaborations from eminent scientists such as René Descartes (1596–1650), Gottfried Wilhelm Leibniz (1646–1716), Henry De la Beche (1796–1855), Elie de Beaumont (1798–1874), William Thomson – Lord Kelvin (1824–1907), James Dana (1813–1895), and Eduard Suess (1831–1914).
In this context, Eduard Suess formulated one of the most critical concepts in stratigraphy, which deals with the cyclicity of global sea level. According to Suess (1888), the contraction of the planet produced eustatic movements. Such movements can be negative (decrease in global sea level) due to the subsidence of ocean basins or positive (increase in global sea level) due to the continuous discharge of sediments that fill these basins. After Suess (1888), a tremendous scientific effort was initiated to understand the planet's internal dynamics, its relationships with the development of ocean basins and eustatic variations, and the potential to use the oscillations of the absolute sea level for global stratigraphic correlations.
In 1890, Grove Karl Gilbert (1834–1918) recommended using the term “diastrophism” to describe the vertical movements of the lithospheric crust. Gilbert (1890) proposed dividing dystrophic processes into orogenic processes, related to the relatively smaller scale that produced the mountain ranges, and epirogenic processes, related to the broader movements that form the boundaries of continents and oceans.
For many years afterwards, the nature of diastrophism was up for debate in the scientific community. “Have diastrophic movements been in progress constantly, or at intervals only, with quiescent periods between? Are they perpetual or periodic?” (Chamberlin, 1909, p. 689). Defending the periodic conception of diastrophism, Thomas Chamberlin (1843–1928) proposed a model for eustasy very similar to Suess (1888), in which the isostatic balance would promote vertical adjustment cycles in the Earth's crust, leading to marine regressions and transgressions. The novelty offered by Chamberlin (1898) was the linkage between diastrophism, sea-level variations, and climatic cycles. In his theory, the weathering of the subaerially exposed continents during regression would promote substantial CO2 consumption, causing global cooling. Conversely, during transgression, the excess of atmospheric CO2 was supposed to improve warming by the greenhouse effect. Chamberlin's primary motivation was to establish a theoretical framework that could explain the global division of geological time and the stratigraphic correlations through base-level changes (Chamberlin, 1909). In his most famous work, Diastrophism as the Ultimate Basis of Correlation, Chamberlin (1909) reaffirms the global character of dystrophic movements and underlines their importance for correlations by base level. According to him, the synchronicity of these events, associated with variations in sea level, allows for transoceanic correlations.
During this same period, William Morris Davis (1850–1934) developed a geomorphic cycle theory to explain landform evolution. According to Davis (1899, 1922), after an initial and rapid tectonic uplift, landforms undergo weathering and erosion processes, evolving through several intermediate stages until culminating in a general peneplanization. A change in the erosion level caused by a new tectonic uplift would cause landform rejuvenation, starting a new geomorphic cycle. Although later criticized for not considering all the complexity of geomorphological processes, Davis's theory became paradigmatic until the mid-20th century. Its cyclical conception influenced ideas about periodic variations in the generation, supply, and preservation of sedimentary deposits.
Barrell (1917) pioneered the understanding of the cyclic behaviour of erosion and accumulation processes. He was the first to propose a systematic link, at different orders, between base-level changes and the preservation of the stratigraphic record. A synthesis of his ideas is presented in the diagram in Fig. 7. With the alternation between deposition and erosion, produced by the harmonic of long-term (diastrophic) and short-term (climatic) base-level fluctuations, Barrell illustrated that most of the geological time is contained in and represented by unconformity surfaces, which he called “diastems”. It is remarkable how many of the principles developed by this author are still in use. The sinusoidal representation of the base-level harmonic oscillations introduced a widespread way of illustrating the logic of stratigraphic evolution (e.g. Van Wagoner, 1990).
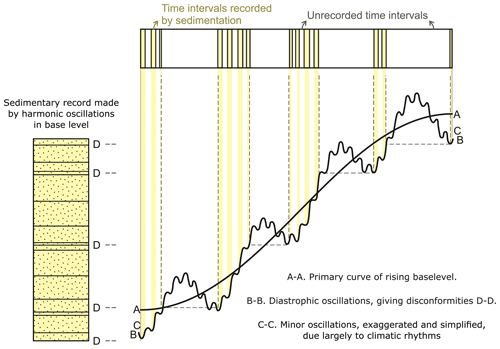
Figure 7Cyclical variations of the base level and their control on preserving the stratigraphic record through an alternation of deposition and erosion (modified from Barrell, 1917).
A year after the First World War, Alfred Wegener (1880–1930) published the first edition of The Origin of the Continents and Oceans. Wegener (1915) was not the first to postulate the lateral movement of continents. However, he deserves the central role in this theme above all for his persistence in defending continental drift against a scientific community hostile to these ideas. The exaggerated reactions to Wegener's theory are due, in part, to the fact that he did not have a satisfactory explanation for the mechanism controlling continental movements (Beckinsale and Chorley, 2003). Another understandable reason is resistance from the scientific community to some theoretical innovations. The continental drift proposal completely contradicted all formulations in force at the time. Since the beginning of the 19th century, what had been advocated in force until the 1960s were the large vertical movements of the Earth's crust, which reached a final formulation in the geosyncline theory (Gnibidenko and Shashkin, 1970).
Hans Stille (1876–1966) was one of the great geologists of the geosyncline theory. Dedicated to describing the evolution of various geological terrains, Stille (1924) mapped successive unconformities in marine deposits. He interpreted that orogenic processes occurred in global synchrony, producing regressions and transgressions of sea level. This proposal cannot be seen as fundamentally new, but Stille (1924) was a pioneer by drawing up the first eustatic variation curve for the Phanerozoic (Fig. 8a).
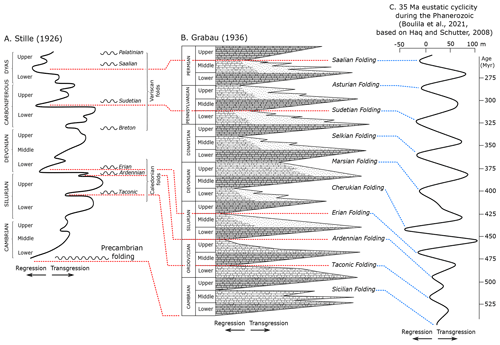
Figure 8Global sea-level curves. (a) Modified from Stille (1926) and (b) modified from Grabau (1936). Both indicate the main orogenetic periods associated with rapid marine regressions. The red lines indicate the same events identified by Stille (1926) and Grabau (1936). (c) Paleozoic eustatic cycles of approximately 35 Myr (determined by bandpass filtering of data presented by Haq and Schutter, 2008) and potential correlation (blue lines) with equivalent cycles of Grabau (1936) (modified from Boulila et al., 2021).
Amadeus William Grabau (1870–1946), through detailed stratigraphic data and correlations in extensive areas of North America, Europe, and Asia, presented a proposal for sea-level fluctuations for long geological periods (Fig. 8b). Although Stille's and Grabau's cyclic conceptions of sea-level variations are similar, Grabau questioned the synchronicity of orogenies in the entire world. He considered these processes to be of local importance and believed that simultaneous sea-level fluctuations could be related to changes in the volumes of ocean basins (Johnson, 1992). Grabau was inspired by the work of Alfred Wegener (Mazur, 2006), and he cited The Origin of the Continents and Oceans in his most significant publication, The Rhythm of the Ages: Earth History in the Light of the Pulsation and Polar Control Theories, published in 1940 (Johnson, 1992).
2.2.2 Plate tectonics and Wilson cycles
Scientific progress and field evidence, particularly concerning the origin of mountain belts, have resulted in the questioning of the contraction theory (e.g. Dutton, 1874), which was finally abandoned. A crisis in the field of tectonics was triggered by the discovery of radiometric dating, which challenged the Earth's long-term cooling, and by the Alpine nappes and thrust sheets that demonstrated the mechanisms of large horizontal displacements of the crust. This crisis did not end until the definition of plate tectonics in the 1960s (O'Hara, 2018).
During the 1960s, advances in post-World War II oceanographic research provided evidence for the evolution of the ocean floor. Such discoveries explained Alfred Wegener's theory of continental drift (Kearey et al., 2009), and the roots of the future plate tectonic paradigm were established (Le Pichon, 2019). The development of this theory can be considered the most significant advance in understanding the Earth's dynamics and has even influenced the study of other planets (e.g. Hawkesworth and Brown, 2018; Karato and Barbot, 2018; Duarte et al., 2021).
John Tuzo Wilson (1908–1993) was one of the leading geoscientists developing the theory of plate tectonics. Wilson (1965) was the first to mention the existence of large rigid plates, describing specific limits of these, which the author called transform faults. However, Wilson's most emblematic work was published the following year. Wilson (1966) presented a specific aspect of the geotectonic process, showing the oceans' successive opening and closing (Fig. 9). Today, the so-called Wilson cycle describes the periodicity with which large continental masses separated and came back together. Over the past 50 years, this concept has proven to be crucial for the theory and practice of geology (Wilson et al., 2019).
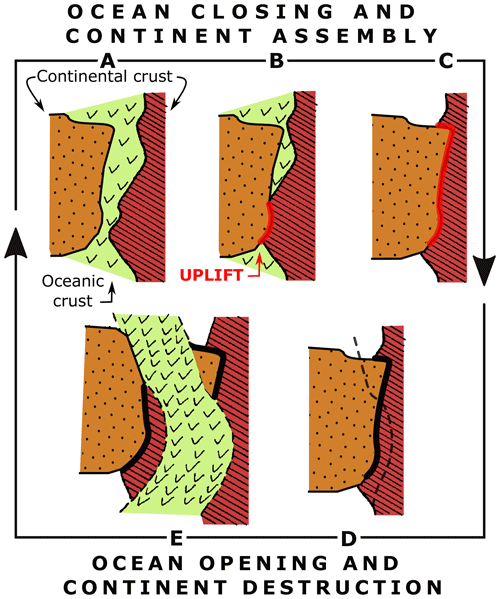
Figure 9Ocean closing and opening cycle (modified from Wilson, 1966). (a) A closing ocean; (b) first contact between two opposite continental coasts; (c) ocean closure and final collision of opposite continental coasts; (d) a hypothetical line (dashed) along which a new continental rupture would engender a younger ocean to re-open; (e) a new ocean opening after the break-up of an old continent.
It is notorious how the theory of plate tectonics followed the stubborn uniformitarianism of processes advocated by James Hutton and Charles Lyell. Stern and Scholl (2010) related the tectonic processes to cycles of creation and destruction of the continental crust, defining a particular equilibrium on Earth. They encapsulated this equilibrium in the traditional Chinese concept of yin–yang, whereby dualities work together and in opposition. About this maintenance of geological systems defined by plate tectonics, Schwarzacher (2000, p. 51) wrote the following.
The environments of deposition from the Precambrian onwards have been similar and repeat themselves; apart from the fortunate exception of the biosphere, there are very few indications of a progressive development in geological processes during the last 1000 Ma. Indeed, based on our present observations, one could easily believe that most sedimentation and therefore stratigraphy should have ended long ago. All basins should have been filled and all mountains eroded. This is not the case and leads us to believe that tectonic events must interfere and revitalize the sedimentation systems.
The Wilson cycle was vital in defining the assembly and the breaking up of supercontinents. This self-organization in plate tectonics has been studied for decades, whose periodicity is in the range of 300–800 million years (Mitchell et al., 2021). Hence, new hypotheses for global cycles could also be formulated, and several questions about the impacts of tectonic events on sea-level and climatic variations were answered. For example, based on the Wilson cycles, Fischer (1981, 1982) formulated the climatic oscillation produced by Earth's icehouse and greenhouse states (Fig. 10).
2.2.3 Internal geodynamic forcings in the Earth system
Currently, the periodicity of several processes in the Earth's internal dynamics is well known (e.g. Matenco and Haq, 2020; Fig. 11). Mitchell et al. (2019) conducted time-series analyses of hafnium isotopes in zircon (Hf-zircon) to identify statistically significant periodicities of magmatic systems throughout geological time. The Hf-zircon analysed by LA-ICP-MS (laser ablation inductively coupled plasma mass spectrometry) represents a well-dated proxy for the evolution of magmatism related to tectonic and mantle convection cycles. From time-series analysis of the global Hf-zircon database for the last ∼ 2 Gyr, the authors defined a hierarchy of geodynamic cycles (Fig. 12), analogous to the orbital ones (Fig. 2).
Mitchell et al. (2019) recognized the periodicity of the superocean cycle (∼ 1.2 Gyr), the supercontinent cycle (∼ 600 Myr), the Wilson cycle (∼ 275 Myr), and an upper-mantle cycle (∼ 60–80 Myr). These cycles appear to be harmonics, implying a coupling between the mantle and lithosphere convections. In addition to these, magmatic cycles of ∼ 20 and ∼ 6 Myr are suggested by the high-resolution circum-Pacific records. According to these authors, “the hierarchy of geodynamic cycles identified with Hf isotopes of zircon appears to represent, according to bandwidth, the last frontier of cyclicity in the Earth system to be identified and explored” (Mitchell et al., 2019, p. 247).
Climatic and eustatic oscillations may have interacted with internal geodynamic processes as triggers or feedbacks (e.g. greenhouse–icehouse cycles; Fig. 10). Changes in ocean circulation related to the configuration of the continents and global volcanic pulses are an example of a potential influence on Earth's climate (Rampino et al., 2021). The link between Earth's internal dynamics and eustasy may come from changes in the volume of marine waters (water exchange with a mantle) and in the volume available in ocean basins (ocean ridge volume; dynamic topography; seafloor volcanism; continental collision), which operate in the long term (greater than 1 Myr; e.g. Sames et al., 2016, 2020; Fig. 13).
Disagreements about the global synchronicity of tectonic cycles have been raised since the beginning of the 20th century. According to Willis (1910, p. 247), “each region has experienced an individual history of diastrophism, in which the law of periodicity is expressed in cycles of movement and quiescence peculiar to that region”. This idea was encapsulated in the concept of relative sea-level change (e.g. Wilgus et al., 1988). Relative sea-level change (as opposed to eustatic sea-level change) is caused by tectonic deformation of the crust in marine and coastal areas, which results in uplift and subsidence of the land relative to the sea surface. Generally, these processes have a local to regional extent and occur at a higher frequency than global geodynamic processes (e.g. Matenco and Haq, 2020; Fig. 11). Thus, sea-level changes caused by geodynamic processes can be local when such processes are also localized (e.g. bradyseism; Fig. 4).
The cyclical behaviour of the mantle and the lithosphere, in association with astronomical cycles, completes the puzzle of cyclicity in the Earth system. The connection between the Earth's internal and external systems is not adequately investigated because tectonic and astronomical influences are often considered independently. Boulila et al. (2021) suggest a potential coupling between Milankovitch forcing and Earth's internal processes for the eustatic sea-level record in the 35 Myr cycle range during the Phanerozoic. This is a cyclicity that is compatible with the one that was recognized a long time ago, by several authors, such as Stille (1926) and Grabau (1936) (Fig. 8c). A challenge for stratigraphy is understanding how the Earth system's conduction mechanisms are imprinted in the geological record. As Barrell (1917) concluded, “sedimentation is controlled by them, and the stratigraphic series constitutes a record, written on stone tablets, of these increasing waves of change that pulsed through geological time”. Such “waves” may correspond to the causal mechanism of biological extinctions, comet impacts, orogenic events, oceanic anoxic events, and sea-level changes, which support the division of geological time into intervals for global correlations (e.g. Rampino et al., 2021; Boulila et al., 2021).
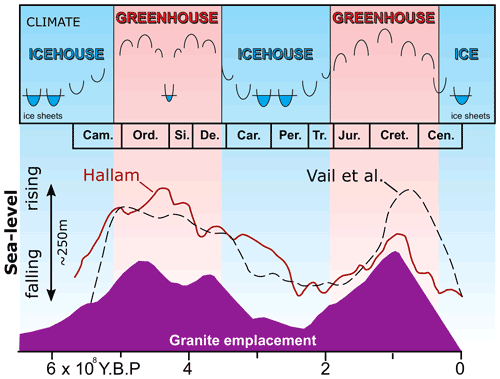
Figure 10Cyclic outlines of Phanerozoic history (modified from Fischer, 1981, 1982). Climatic oscillations are composed of greenhouse and icehouse states, with minor internal climatic fluctuations. Sea-level curves, according to Vail et al. (1977) and Hallam (1977). Global granite emplacement was deduced from data based on the American granite emplacements (after Engel and Engel, 1964).
The idea of a cycle involves repetition because a cycle can be recognized only if units are repeated in the same order. The question that inevitably arises is: How closely similar must the repetition be? An answer seems to depend on two requirements: (1) nearly complete transitions between variants must be observed, and (2) a generalization must be made reducing the cycle to its simplest form by excluding all unessential details. The cycles, then, must be closely similar with respect to this simple form (Weller, 1964, p. 613).
According to Goldhammer (1978), most, if not all, stratigraphic successions exhibit repetitions of strata at different scales. Throughout the history of stratigraphy, the concept of cyclicity played a crucial role in the inductive observations of the record and subsequent deductive reasoning. Several approaches have been used to describe this cyclicity. Among them, the following lines of description and interpretation will be briefly presented: sedimentary facies cycles, cyclothems, clinoforms, stratigraphic sequences, and astrocycles.
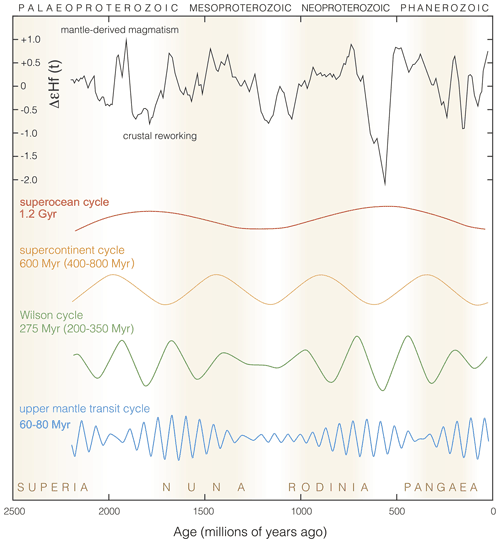
Figure 12Global Hf database (black) and cycles determined by the time-series analysis: superocean cycle (∼ 1.2 Gyr; red), the supercontinent cycle (∼ 600 Myr; yellow), the Wilson cycle (∼ 275 Myr; green), and an upper mantle cycle (∼ 60–80 Myr; blue).
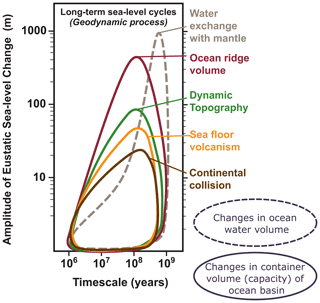
Figure 13Log-scale diagram of the timing and amplitudes of the main mechanisms that control “long-term” sea-level variations related to internal geodynamic processes. The values represented must be considered the average (modified from Sames et al., 2016).
3.1 Sedimentary facies cycles
Sedimentary cycles are recurrent sequences of strata each consisting of several similar lithologically distinctive members arranged in the same order. A great variety of cycles is possible ranging from simple to quite complex but only a comparatively few types actually have been recognized. Cycles may be either symmetrical or asymmetrical depending upon the pattern presented by their members. They record the occurrence of definite series of physical conditions, and resulting sedimentary environments, that were repeated in the same order with only minor variations (Weller, 1960, p. 367).
During the 15th and 16th centuries, observing the landscape and the natural phenomena that modify it played a crucial role in constructing modern science, especially in the Earth sciences (Puche-Riart, 2005). For example, through detailed observations of successive rock strata, Leonardo da Vinci (1452–1519) expressed nature in his paintings (Ferretti et al., 2020). He was probably one of the first to understand erosion, transport, deposition, and lithification processes from field observations. In the Codex Leicester, Leonardo da Vinci shows the vertical and lateral organization of rocky beds observed in the Alps that he interpreted as a record of river flood cycles (Ferretti et al., 2020).
In 1669, Nicolaus Steno (1638–1686) published one of the most crucial works about the genesis of rock layers and their fossil components. Based on an interpretation of the geological evolution of Tuscany, he proposed three fundamental stratigraphic principles that continue to be used today (Kravitz, 2014). Through an evolutionary diagram (Fig. 14), Steno suggested that the sedimentary beds are formed by successive floods, followed by reworking that erodes and deforms them. He noted that sediment layers were deposited in chronologic successions that display the oldest layers on the bottom and the youngest ones on the top of the pile (principle of superposition). According to him, initially, the strata are organized in a set of horizontal layers (principle of original horizontality) that could be later eroded and deformed, and new horizontal layers are deposited over them. Concerning the strata's geometry, Steno defined each sedimentary bed as extending laterally in all directions (principle of lateral continuity) until it reached an obstacle, such as the basin's border.
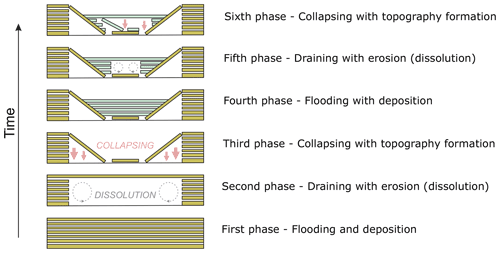
Figure 14Steno's evolutionary diagram describes six stages for the geologic history of Tuscany, including flooding cycles and crustal collapse (modified from Kravitz, 2014).
Nicolaus Steno was responsible for introducing the term “facies” into the geological literature. He used it to describe the fundamental characteristics of a part of the Earth's surface during a specific geological time (Teichert, 1958). Later, this concept evolved through the descriptions of Amanz Gressly (1814–1865) in the Jura mountains at the French–Swiss border. Gressly (1838) defined the sedimentary facies as the different lithological features and fossil components of a sedimentary layer, interpreted as a record of the original depositional processes. He explained the genesis of sedimentary facies as the product of processes that operated in depositional environments and demonstrated, through stratigraphic correlations, the lateral facies transitions that compose a mosaic of environments along a depositional profile (Cross, 1997).
In 1894, Johannes Walther (1860–1937) introduced an essential geological principle associated with the concept of facies (Middleton, 1973). Known as Walther's law of facies, this principle states that any vertical facies succession is a record of depositional environments that were laterally adjacent to each other in the geological past. This vertical and lateral facies correspondence is still used today for paleogeographic reconstructions, especially when associated with an actualistic approach (e.g. Fragoso et al., 2021).
Between the 19th and 20th centuries, several works presented detailed sections demonstrating repeated associations of different types of rocks (Weller, 1964). The economic interest in carboniferous coal beds fueled some of the earliest observations. In 1912, Johan August Udden (1859–1932) was a pioneer in recognizing cycles in the stratigraphic record. In a report about the geology of the US state of Illinois, he identified facies cycles in Pennsylvanian strata, composed, from bottom to top, by layers of coal, limestone, and sandstone (Fig. 15). Udden (1912) interpreted such cycles as products of successive transgressions and regressions of the shoreline during the basin's subsidence. He established that stratigraphic surfaces marked by paleosols correspond to the end of each cycle. According to him, these surfaces represent depositional gaps.
Laboratory simulations were introduced during the 1950s and 1960s, culminating in the flow regime concept (Simons and Richardson, 1966). This advance improved the interpretation of sedimentary structures preserved in the geological record (e.g. Allen, 1963; Middleton, 1965). Concomitantly, there was also much progress in facies models through studies of modern sedimentary environments (e.g. Fisk et al., 1954; Illing, 1954; Oomkens and Terwindt, 1960; Bernard and Major, 1963; Shearman, 1966; Glennie, 1970).
In the 1960s, the stratigraphic application of facies models evolved considerably through the analysis of cyclicity seen in the outcrops (e.g. Weller, 1960). Recurrent sequences of sedimentary facies, arranged in a specific order, have been interpreted as the record of similar depositional and environmental processes, repeated at all scales, from millimetres to many hundreds of metres (Goldhammer, 1978; Schwarzacher, 2000). In this context, specific terms were created for describing sedimentary facies with regular alternation, such as “cyclites” or “rhythmites” (e.g. Kvale, 1978; Brodzikowski and Van Loon, 1991). Although generic, these terms have been closely associated with regular climate cycles (e.g. Chandler and Evans, 2021) or those produced in tidal environments (e.g. Kvale, 1978).
Researching cyclic depositional mechanisms in alluvial plains, Beerbower (1964) defined the concepts of autocyclic versus allocyclic. Autocyclic was defined as the sedimentation record generated purely within the given sedimentary system by the distribution of energy and sediments, such as lateral channel migration and meander abandonment. On the other hand, allocyclic was associated with the external processes that cause changes in the alluvial channels' discharge, loading, and inclination. They differ from autocyclic alternations in their wider lateral extension along the basin or even to other depositional basins.
With some modernizations, the concepts of autocyclic and allocyclic controls currently encompass all geochemical, ecological, and physical sedimentary processes (Cecil, 2003). Nowadays, autocyclic dynamics are understood as the spontaneous form of deposition within sedimentary systems, determining spatial and temporal heterogeneities in the way sediments and water are distributed in a landscape (Hajek and Straub, 2017; Fig. 16). Delta switching and lateral migration of channels, dunes, or ripples are examples of autocyclic processes that produce cyclical deposits (e.g. Hajek and Straub, 2017; Miall, 2015). Other examples include episodic events, which, although recurrent, do not have periodicity, such as storms and sediment gravity flows (e.g. Einsele, 2000). The autocyclic dynamics must be self-regulating and include feedback mechanisms to produce cyclic sedimentary records (Goldhammer, 1978). Since they do not always have a periodic regularity, the preference is to use the term “autogenic” (Miall, 2016).
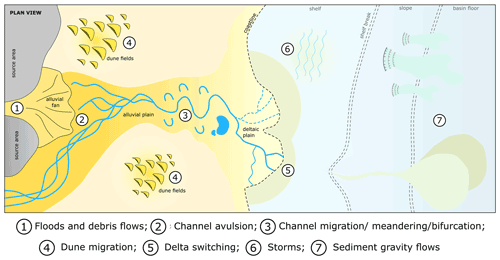
Figure 16Schematic illustration with some autogenic controls on sedimentation in different environments.
In turn, allocyclic (or allogenic) controls correspond to regional or global processes fundamentally related to climate, eustasy, and tectonics. These processes influence, at different magnitudes and frequencies, the production, transport, accumulation, and preservation of sediments, be they inorganic or organic, clastic, or chemical (e.g. Strasser et al., 2006; Holbrook and Miall, 2020; Matenco and Haq, 2020; Fig. 17). In contrast to autocycles, the allocyclic controls are regular and tend to have known frequencies (as seen in Sect. 2). They also define accommodation (defined by eustatic sea level and subsidence) and make the link to sequence stratigraphy (e.g. Holbrook and Miall, 2020; Fragoso et al., 2021). Hilgen et al. (2004) advised that even the record produced by sudden autocyclic events (e.g. storms) may occur in clusters related to allocyclic controls (e.g. astronomical). Furthermore, the understanding of the organization of fluvial systems, mainly controlled by the autogenic dynamics, was discussed by Abels et al. (2013). According to these authors, the regularities in such systems could be linked to allogeneic, astronomically forced climatic changes.
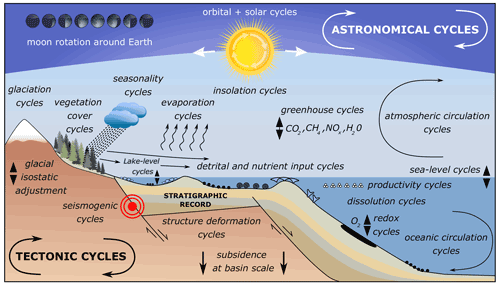
Figure 17Schematic diagram illustrating the main allocyclic controls on sedimentation (modified from Strasser et al., 2006).
Over the years, several authors raised the question of how sedimentary preservation influences and possibly hampers the analysis and interpretation of facies and stratigraphic organization.
What does the stratigraphic record actually record? This rather fundamental question spawns more questions, all of which are building blocks in the foundations of geology. Are the processes and events recorded in the rocks truly representative of their time? At what resolution do rocks record processes? What determines which examples of a repeated process are actually preserved? What is missing? What can be determined with certainty from what remains? Geologists have mulled the answers to these questions at various intensities since geology was in its infancy. The answers to these questions ultimately determine the legitimacy of every interpretation made of the past from the stratigraphic record (Holbrook and Miall, 2020, p. 1).
Barrell's (1917) proposal for the alternation of deposition (base-level rise) and erosion (base-level fall) processes at multiple amplitudes and frequencies (Fig. 7), in which only one-sixth of the time is preserved in the rock record, illustrates this question in a precise way. It is concluded that much of geologic time is distributed across numerous gaps in the record (e.g. Dott, 1983; Udden, 1912; Ager, 1993; Sadler, 1999; Miall, 2015; Strasser, 2015; Holbrook and Miall, 2020), which limits the use of Walther's law of facies in reconstructing laterally adjacent paleoenvironments (Fragoso et al., 2021).
In this respect, within what is considered “sedimentary geology” (sensu Middleton, 1978), there is a difference between sedimentological analysis, which is concerned with interpreting the processes at the origin of sedimentary facies, to stratigraphic analysis, which is mainly related to the organization of facies in geological time. With certain poetic freedom, it would be like considering that the harmonic amplitudes and frequencies of the base level oscillations compose the stratigraphic “music”, producing sedimentary “notes” spaced in time. Furthermore, as Wolfgang Amadeus Mozart said, “the music is not in the notes, but in the silence between”. For this reason, stratigraphers must pay as much attention to surfaces that contain the gaps as they do to sedimentary facies, taking into account the effect of preservation.
Miall (2015), Holbrook and Miall (2020), and Miall et al. (2021) encapsulated this thought in a more objective and mechanistic way through the concept called a “preservation machine” or “stratigraphy machine” (Fig. 18a). These authors considered that the organization of the stratigraphic record occurs through multiple overlapping of autogenic and allogeneic processes, which generate and remove sedimentary deposits across the whole range of geological timescales. Furthermore, the “cycles to preserve” (i.e. the number of sedimentary cycles needed to ensure some preservation at a given scale) constitutes a part of the rock record at each timescale, which can potentially be analysed hierarchically (Fig. 18b).
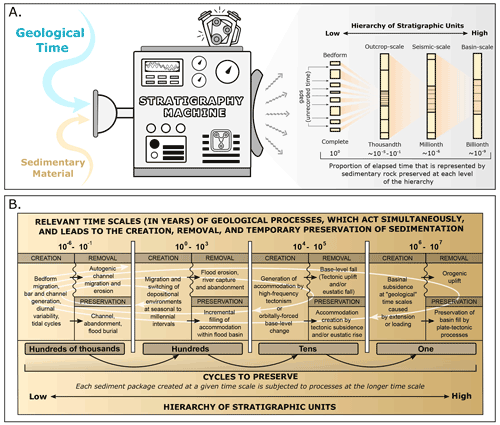
Figure 18Stratigraphy machine. (a) Playful representation of the “stratigraphy machine” concept that generates the stratigraphic record, organizing geological time into hierarchically preserved sedimentary units and hiatus surfaces from the bedform to the entire basin fill (based on Holbrook and Miall, 2020). (b) Table illustrating the stratigraphy machine's operation, which considers the simultaneous action of several accumulation, removal, and preservation processes, which interact at different timescales to generate the rock record. For convenience, the timescale is subdivided into four broad intervals. The diagram should be read from left to right, where at each time interval the sediments are first generated by the depositional processes, and what is not removed on the surface is preserved in the subsurface, creating the initial succession. Over time, long-term processes affect this succession, with preservation and/or removal. In this way, long-term processes will affect short-term processes, as indicated by the loops at the bottom of the figure. It is estimated that a period equal to or greater than 107 years would be enough for all processes to perform a complete cycle. Due to the recurrent removal processes, numerous sedimentary gaps occur in the final product at all scales, and the rock record represents only a fraction of the elapsed time (modified from Holbrook and Miall, 2020).
3.2 Cyclothems
Between the 1930s and 1960s, the sections presented by Udden (1912) became emblematic. Initially called “suites” (Wanless, 1929) or “cyclical formations” (Weller, 1930; Wanless, 1931), it was the term “cyclothems” (Wanless and Weller, 1932) that triumphed in the literature for describing such cyclic facies alternations.
The concept of cyclothems has become familiar to most geoscientists who describe sedimentary facies repetitions (e.g. Weller, 1943). The progress of the work in the Pennsylvanian of Illinois revealed that the recurrence of individual cyclothems not only corresponds to the unique rhythms to be observed in stratigraphic successions, but is also part of a larger order.
This repeated succession of cyclothems of different character indicates a rhythm of larger order than that shown in the individual cycles and suggests the desirability of a term to designate a combination of related cyclothems. The word “megacyclothem”” will be used in this sense to define a cycle of cyclothems (Moore et al., 1936, p. 29).
According to James Marvin Weller (1899–1976), “these larger rhythms may be the long-sought key that will solve some of the perplexing problems of interbasin correlation” (Weller, 1943, p. 3). This author later proposed the existence of even larger groups, called hypercyclothems (Weller, 1958). This marked characteristic of the cyclicity in the sedimentary record, in which individual cycles occur in clusters that make up larger cyclical units, remains in modern approaches of sequence stratigraphy (Catuneanu, 2019a, b; Magalhães et al., 2020; Fragoso et al., 2021; see item 3.3) and cyclostratigraphy (e.g. Hinnov, 2018; see item 3.4) The term “stacking pattern” is often used to describe a hierarchical order of cyclical units.
Raymond Cecil Moore (1892–1974) presented another feature of the cyclical stratigraphic record quite pertinent in the modern context of sequence stratigraphy, concerning the definition of boundary surfaces. According to Moore (1964), both cyclothems and megacyclothems are limited by key surfaces, marked by disconformities or a change from continental to marine sedimentation (Fig. 19).
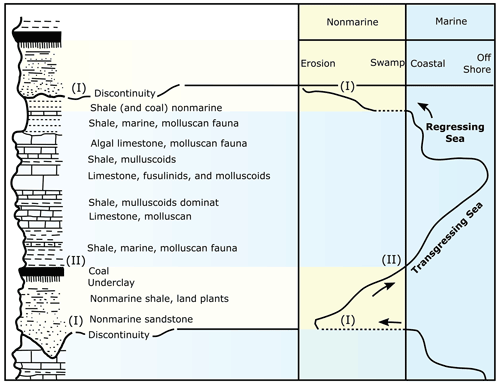
Figure 19Representative section of cyclothems indicating the alternation of continental and marine paleoenvironments (modified from Moore, 1964). The alternatives of limits for cyclothems are (I) disconformities and (II) the transition from non-marine to marine conditions.
Concerning the origin of cyclothems, Klein and Willard (1989) argued that such units are the product of the combined action of tectonic and eustatic processes. According to these authors, the integrated analysis of parameters related to geotectonic evolution, global paleoclimate (controlled by orbital, Milankovitch cycles), and laterally changing regional subsidence allows understanding the paleogeographic variations that gave rise to marine and continental cyclothems, along with lateral correlations (Fig. 20). This approach presents many parallels to the analysis of systems tracts in the context of sequence stratigraphy (e.g. Posamentier et al., 1988; Hunt and Tucker, 1992; Posamentier and Allen, 1999).
3.3 Clinoforms
A broader analysis of the geometry of sedimentary deposits also revealed sedimentological alternations, which contributed to the definition of cyclic stratigraphic units. John Lyon Rich (1884–1956) was the first to describe the inclined geometry of marine deposition. Rich (1951) defined that, along a transect from coast to basin, the sedimentary deposits can be subdivided into three depositional forms: undaform, clinoform, and fondoform (Fig. 21). Among these terms, only “clinoform” is being used nowadays. However, the theoretical basis brought by such an approach remains similar, especially regarding the possibility of shifts between these environments caused by sea-level changes (Fig. 21b), resulting in characteristic successions of the geometry of strata (Fig. 21c).
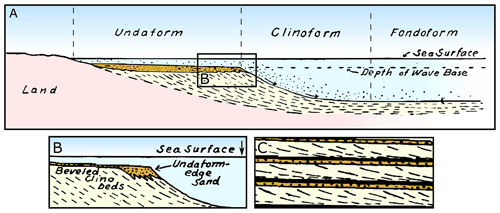
Figure 21Sketches and terminology for coastal marine deposits (modified from Rich, 1951): (a) undaform, clinoform, fondoform. (b) Area of thick sand on the outer edge produced by the slight reduction in sea level. (c) Alternations of coastal marine deposits produced by intermittent changes in sea level.
DeWitt Clinton Van Siclen (1918–2001) considered the sloping geometries of continental margin deposits to describe the lateral variations observed in the cyclothems. According to Van Siclen (1958), the alternation of fluvial and coastal deposition with erosional disconformities predominates landward, grading basin-ward to alternating marine and terrigenous deposition and finally reaching a totally marine domain, with an alternation of clastic and carbonate deposits. The author described cycles in the deep sea composed of clastic sedimentation during stable or lowered sea level and non-deposition or thin black-shale layers deposited during higher sea stands. Considering different scenarios of changes in sea level and sediment supply, Van Siclen (1958) proposed distinct types of clinoform successions (Fig. 22). This approach was handy for correlating well data when seismics did not support the oil and gas industry. It is interesting to realize how such a concept is similar to the current sequence-stratigraphic models.
3.4 Stratigraphic sequences
Stratigraphic cyclicity can be observed at different scales. At each scale of observation (i.e., hierarchical level), the building blocks of the sequence stratigraphic framework are represented by sequences and their component systems tracts and depositional systems (Catuneanu, 2019b, p. 128).
Laurence Louis Sloss (1913–1996) is widely recognized as one of the pioneers of the concept of sequence stratigraphy, and many credit him with instigating a revolution in stratigraphic thinking (Dott, 2014). Sloss et al. (1949) used for the first time the term “sequence” to refer to stratigraphic units that could be correlated over large areas through geological mapping and well data. Subsequently, this sequence model defined successive stratigraphic units bounded by “interregional unconformities” that covered the North American craton (Sloss, 1963; Fig. 23).
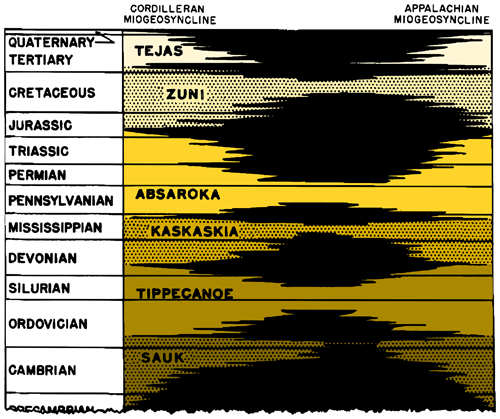
Figure 23Sequences of the North American craton (modified from Sloss, 1963). The black areas represent temporal gaps, and the light areas represent the depositional units.
In the late 1960s, under Sloss' guidance, Peter Vail, Robert Mitchum, and John Sangree studied North American Pennsylvanian cyclothems (Dott, 2014). Similarly to small-scale versions of Sloss sequences, bounded by numerous widespread unconformities, these cyclothems were interpreted by them as the stratigraphic record of glacioeustatic fluctuations. Subsequently, these three geologists collaborated with the Exxon research group to develop the method of interpreting seismic data, refining their mentor's concept of sequence (e.g. Mitchum, 1977).
During the 1960s and 1970s, the evolution of seismic interpretation was responsible for reuniting many stratigraphic concepts that underlie the current sequence-stratigraphic methodology. The first reference to the term “seismic stratigraphy” was published at the 27th Brazilian Congress of Geology (Fisher et al., 1973), and efforts in this area gained prominence in the international community through AAPG Memoir 26 (Payton, 1977), where the main techniques developed by the Exxon research group were presented. The great innovation was to consider the continuous reflectors observed in seismic sections to be depositional timelines. In this way, it became possible to interpret that surfaces representing an unconformity pass laterally to a correlative conformity, which was fundamental for the definition of a sequence (e.g. Mitchum, 1977). The seismic interpretation, together with biostratigraphic constraints, made it possible to establish chronostratigraphic correlations within a basin and between different basins (e.g. Mitchum and Vail, 1977; Fig. 24). According to Vail (1992), this approach aimed at providing a unifying concept for sedimentary geology equal to what plate tectonics had done for structural geology.
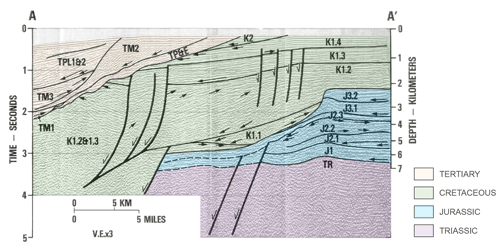
Figure 24Seismic section from offshore north-western Africa showing sequences defined by seismic reflectors. Black lines show the sequence boundaries (modified from Mitchum and Vial, 1977).
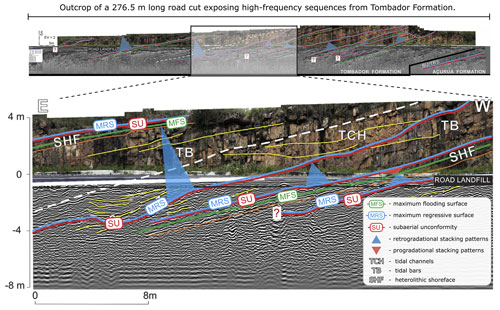
Figure 25High-frequency sequences identified in an outcrop and in a ground-penetrating radar (GPR) profile of the Tombador Formation (Mesoproterozoic), Chapada Diamantina region, Brazil (modified from Magalhães et al., 2017). The sequences are composed of tidal channels and bars, bounded at the top by heterolithic intervals that configure cycles of retrogradational stacking patterns defined by the recurrence of the same type of stratigraphic surface in the geological record.
Different sequence-stratigraphic models were presented between the 1970s and 1990s, resulting in a profusion of concepts and jargons. Catuneanu (2006) offered a complete review of these proposals. After the 2000s, a scientific effort was made to standardize the nomenclature and the methodology of sequence stratigraphy (Catuneanu et al., 2011), defining a simple and integrating workflow appropriate for modern stratigraphic analysis (Miall, 2016).
Over time, sequence characterization has proven helpful in academic and industrial applications since such units constitute a natural structure for classification and local to regional correlations (e.g. Fragoso et al., 2021). Catuneanu and Zecchin (2013; p. 27) defined sequences as a “cycle of change in stratal stacking patterns, dividable into systems tracts and bounded by sequence stratigraphic surfaces”. The current sequence-stratigraphic methodology has a scale-independent approach, in which sequences can be defined from the basin (sensu Sloss et al., 1949; Sloss, 1963) to facies scale (e.g. Strasser et al., 1999; Magalhães et al., 2016, 2017; Fig. 25), ordered in a hierarchical framework (Magalhães et al., 2020).
According to Fragoso et al. (2021), the characterization of sequences within a cyclic and hierarchical framework should obey the following criteria (Fig. 26): transgressive–regressive (T–R) cycle anatomy; vertical recurrence of stacking patterns; vertical trends in the stacking patterns composing subsequent hierarchies of cyclicity; recognizable mappability. In this sense, a stratigraphic sequence framework is composed of cycles observed at different hierarchies. A higher ranking comprises an organized cluster of lower-ranking sequences (Catuneanu, 2019a, b; Magalhães et al., 2020; Fragoso et al., 2021; Fig. 27). This cyclic approach of the stratigraphic analysis supports the objective results in predicting the vertical recurrence and the lateral correlation of genetic stratigraphic units.
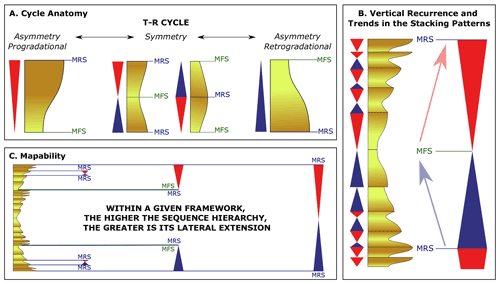
Figure 26Characteristics and criteria for defining stratigraphic sequences within a cyclic and hierarchical framework (modified from Fragoso et al., 2021): (a) T–R cycle anatomy. (b) Vertical recurrence of individual cycles and trends in the cyclic stacking pattern (modulation of the smallest by the highest hierarchy). (c) Mappability of the stacking patterns and stratigraphic surfaces within a given framework, which is more significant the higher the hierarchy is. Abbreviations: MFS – maximum flooding surface; MRS – maximum regressive surface.
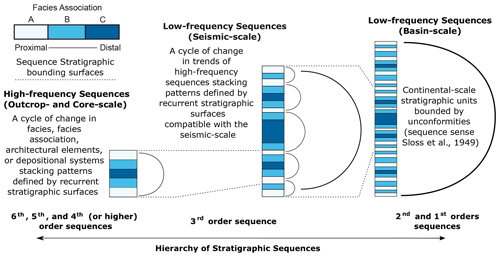
Figure 27Hierarchical stratigraphic sequence framework (modified from Magalhães et al., 2020). High-frequency sequences (fourth or higher orders) are observed at outcrop and core scale. The vertical recurrence of high-frequency sequences composes the low-frequency sequences observed in seismic data (third order). First- and second-order low-frequency sequences occur at basin scale predominantly limited by unconformities, as proposed by Sloss et al. (1949).
3.5 Astrocycles
Gilbert (1895) was the first to consider that the sedimentary record may exhibit repetitions controlled by orbital cycles. He correctly suggested that the Upper Cretaceous marl–limestone alternation in the US state of Colorado should correspond to an allocyclic record of climatic oscillation controlled by the orbital precession cycle of about 20 kyr. Although rudimentary, Gilbert's conclusions allowed the measurement of geological time using the sedimentary record before the invention of radiometric dating (Strasser et al., 2006). After Gilbert, the studies of astronomically forced climatic cycles evolved considerably from Adhémar (1842), Croll (1875), and, especially, Milankovitch (1941). The application of this knowledge to sedimentary successions emerged gradually.
In the 1960s, some studies started identifying cycles in different depositional contexts related to orbital forcing. For example, Van Houten (1964) presented the cyclic character of the lacustrine record of the Upper Triassic Lockatong Formation in the United States. This work stands out by determining a stratigraphic ordering in three hierarchies and proposing a temporal definition based on orbital cycles (Fig. 28).
In 1976, one of the most influential articles in the study of Milankovitch's theory was published. In their work entitled “Earth Orbit Variations: The Ice Age Pacemaker”, James Hays, John Imbrie, and Nick Shackleton established the effects of orbital parameters on the long-term climate record obtained from the analysis of marine sediments. Thus, Hays et al. (1976) “legitimized what was to become one of the most powerful tools in stratigraphy” (Maslin, 2016, p. 208).
In the 1980s, the studies about the geological record of astronomical cycles integrated a subdiscipline of stratigraphy named “cyclostratigraphy” (Strasser et al., 2006). According to Hilgen et al. (2004), cyclostratigraphy identifies, characterizes, correlates, and interprets cyclical variations (periodic or quasi-periodic) in the stratigraphic record. In cyclostratigraphic studies, temporal calibrations can be done by either correlating sedimentary cycles – identified through variations in paleoenvironmental or paleoclimatic proxies sampled along a section or core (e.g. Li et al., 2019) – or by astronomical target curves of precession, obliquity and eccentricity, or by related insolation curves (Strasser et al., 2006). Weedon (2003) and Kodama and Hinnov (2015) present mathematical techniques for processing signals obtained by these proxies. Once the periodicity of a sedimentary cycle has been demonstrated, a very detailed analysis of sedimentological, paleoecological, or geochemical processes can be evaluated in a high-resolution time-stratigraphic framework (Strasser et al., 2006).
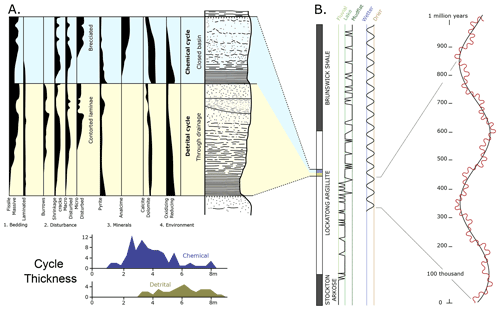
Figure 28Cyclic lacustrine sedimentation of the Upper Triassic Lockatong Formation (modified from Van Houten, 1964). (a) Model of detrital and chemical short cycles; (b) generalized stratigraphic section of Lockatong Formation and adjacent units. The columns show the alternating geographic environments and the long climatic cycles of wetter and drier phases. An age model is presented based on two long climatic cycles, with intermediate and short cycles associated.
The term “sedimentary cycle” in cyclostratigraphy has a specific meaning, which differs from more generic applications (e.g. Weller, 1960). The sedimentary cycle as used in cyclostratigraphy corresponds to “one succession of lithofacies that repeats itself many times in the sedimentary record and that is, or is inferred to be, causally linked to an oscillating system and, as a consequence, is (nearly) periodic and has time significance” (Hilgen et al., 2004, p. 305; Fig. 29). Thus, Strasser et al. (2006) proposed the term “astrocycle” to define specific cycles whose periodicity can be demonstrated by the cyclostratigraphic analysis.
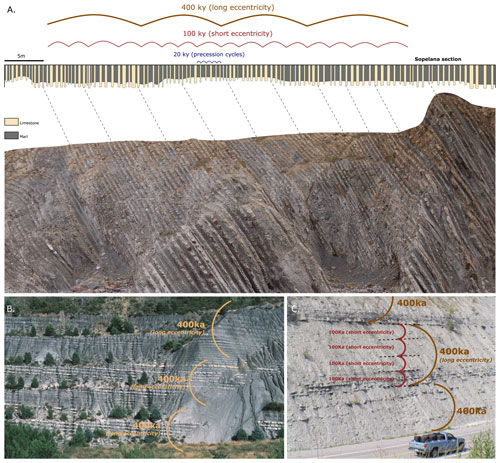
Figure 29Outcrop examples of sedimentary cycles determined by cyclostratigraphic analysis. (a) Long eccentricity, short eccentricity, and precession cycles in hemipelagic limestone and marl alternations of the Sopelana section (Maastrichtian), Spain (modified from Batenburg et al., 2014); (b) long eccentricity cycles in hemipelagic limestone and marl alternations of the La Marcouline section (Middle Aptian), France (modified from Kuhnt and Moullade, 2007); (c) long and short eccentricity cycles in shallow-marine deposits of the Kope Formation (Ordovician), United States (modified from Ellwood et al., 2013).
At this time, cyclostratigraphic analysis is part of integrated stratigraphy, which combines several stratigraphic subdisciplines (e.g. biostratigraphy, magnetostratigraphy, chemostratigraphy, geochronology) to solve problems related to geological time (Hilgen et al., 2015). This integration aids paleoenvironmental interpretation, focusing on multi-proxy analyses, and provides accurate geochronological information for astronomical tuning of stratigraphic records into target curves of orbital cycles and the related insolation curves. Thus, the integrated stratigraphy supports the construction of a high-resolution astronomical timescale that is currently decisive to determine a Global Stratotype Section and Point (GSSP – e.g. Lirer and Laccarino, 2011) and to refine the Geological Time Scale (Gradstein et al., 2021).
Since the beginning of their existence, humans have dealt with cycles. From the simple day–night, hungry–satisfied, and sleeping–awake to the passing of the seasons and the coming and going of migratory animals, cycles are omnipresent and contribute to shaping the human way of thinking. This aspect has had an epistemological influence on observing and interpreting the most diverse natural phenomena that control the Earth system. In Earth sciences, cycle concepts improved geological knowledge, offering simple analytical solutions to describe rock records and interpret geological processes. There is a primordial function in the practice of geology within what is considered a hermeneutic circle (e.g. Frodeman, 1995; Miall, 2004; Frodeman, 2014). This point of view establishes that geology is developed by the processes of induction and deduction, where the set of detailed descriptions supports general theories, while deductive reasoning enhances and refines the descriptive methodologies and techniques (Fig. 30).
Geology is a prominent example of a synthetic science, combining a variety of logical techniques to solve its problems. The geologist exemplifies Lévi-Strauss's bricoleur, the thinker whose intellectual toolbox contains a variety of tools that he or she selects as appropriate to the job at hand (Frodeman, 2014, p. 77).
In the Earth sciences, understanding the entire geological record starts with a primordial rock cycle, in which sedimentary processes are a fundamental part. The cyclic nature of the sedimentary processes is evidenced by multiple steps of erosion–transport–sedimentation experienced by any sedimentary particle from its source rock to its destination in a sedimentary basin. Many organisms also produce sediment, and their life cycles are controlled by cyclically changing environmental conditions. A harmonic produced by oscillations from different sources, frequencies, and amplitudes throughout this long sedimentation process modulates the final sedimentary product. Thus, the cyclical conception has an important implication for understanding the sedimentary record over geological time. In the big picture, the analysis of cyclicity is a crucial tool to correctly decode the sedimentary record (e.g. Barrell, 1917).
However, according to Miall (2004), contrary to the hermeneutic circle, the practice of stratigraphy throughout history has separated the empirical descriptive (inductive) and theoretical (deductive) approaches. Despite the advances and contributions of different research groups working along each of these lines, this author warns of the danger in the use of stratigraphic models that specifically seek evidence of regularity or cyclicity of processes on Earth, which may lead to a bias.
There is no place for superimposed, inadequately grounded theoretical assumptions in the construction of the geologic timescale. The hermeneutic circle is, after all, a circle. We need to be as adept at climbing the upward-directed arrow of theory based on rigorous observation as we are skilled at avoiding the downward slide of making our observations fit our deductions (Miall and Miall, 2004, p. 44).
On the opposite side, but in a complementary way, stratigraphic analysis cannot be reduced to a mere collection of sedimentological descriptions, as gaps span an equivalent or even longer time than the sedimentary rock record (e.g. Dott, 1983; Ager, 1993; Miall, 2004, 2017). Given the lack of tools or parameters for measuring these time gaps in the stratigraphical record, Fragoso et al. (2021) warn that conceptual models are traditionally constructed from a mistaken perspective that assumes the complete preservation of three-dimensional depositional systems based on an oversimplification of continuous sedimentation processes. To guarantee realistic representations, these authors propose that conceptual models incorporate knowledge of the processes that control the generation, destruction, and preservation of sedimentary units to define, at any timescales, predictable and ordered stratigraphic patterns for both the gaps and the preserved record.
Historical perspective is essential for identifying unsolved problems and developing future research programmes. As can be seen throughout this brief review, the identification and interpretation of cycles correspond to a keystone in the history of stratigraphy. However, the use of the term “cycle” has changed through time. Stratigraphic cycles have been described as physical units with characteristic repetitive patterns but not always with a strict periodicity. Facies cycles, cyclothems, clinoforms, and stratigraphic sequences are examples where what is called a “cycle” is a sedimentary unit that repeats itself in the stratigraphic record, with or without inference of the processes responsible for this repetition. In these cases, the concept of cyclicity has a cognitive and didactic purpose, functioning as an association of general ideas that support the description and characterization of the repetitive stratigraphic record. What is interesting to note is that despite the different approaches and nomenclatures, stratigraphic cycles have been described with very similar characteristics, such as stacking patterns, bounding surfaces, and hierarchical frameworks. This common thread of the different approaches paves the way for integrating efforts and the consequent methodological improvement.
For many years, efforts have been made to develop mathematical and statistical tools to characterize stratigraphic cycles. Statistical distribution fitting (e.g. Pantopoulos et al., 2013), Markov chains (e.g. Krumbein and Dacey, 1969; Carr, 1982; Purkis et al., 2012), Fischer plots (e.g. Fischer, 1964; Read and Goldhammer, 1988; Husinec et al., 2008), time-series analysis (e.g. Schwarzacher, 1975; Hinnov and Park, 1998; Weedon, 2003; Martinez et al., 2016), and automatic stratigraphic correlations (e.g. Nio et al., 2005; Behdad, 2019; Shi et al., 2021) are examples of techniques used in stratigraphic research for quantifying cycles. With the so-called digital transformation currently in force in many areas of knowledge, such quantitative approaches tend to be expanded. Thus, the knowledge acquired about the main cyclic characteristics observed in the sedimentary record over the past few years should be the plumb line towards a digital revolution within stratigraphy.
The effort to obtain mathematical solutions is legitimate and perhaps the only way to resolve which cycles (physical observation) are candidates for being periodic (with a predictive time value) or merely episodic. However, the mathematical solutions must be combined with a rigorous analysis of the rock record that independently characterizes the sedimentological, palaeontological, and geochronological aspects. In this sense, integrated stratigraphy is undoubtedly the appropriate way to reinforce the links between different methodologies. Astronomical calibration of the stratigraphic record is appropriate for reducing uncertainties regarding interpretations of changes in sea-level, hydrodynamics, climate, physical, chemical, and biological processes (Schwarzacher, 2000; Hilgen et al., 2004; Strasser et al., 2006; Fragoso et al., 2021).
The recognition of multi-scale stratigraphic cycles, associated with temporal calibrations that better define the relationship – simple or complex – of cause (geological process) and effect (observable stratigraphic entity), will undoubtedly boost the current three-dimensional simulations of depositional systems. In this stratigraphic forward modelling, such parameters have already been used to simulate the genesis of low- to high-frequency sequences in three-dimensional models applied to oil and natural gas exploration and production projects (e.g. Huang et al., 2015; Faria et al., 2017).
In order to deeply understand the cyclicity in geological process, it is necessary to consider its ultimate root: thermodynamics (e.g. Richet et al., 2010). The first law of thermodynamics, also known as the law of conservation of energy, states that energy cannot be created or destroyed but can change from one form to another. In this sense, everything in the Universe can be classified as a form of energy, regardless of its physical nature. Thus, it is possible to convert energy into any different form, be it a rock, a tree, or a human being. When we consider the Law of Conservation of Energy applied to deep time, it becomes possible to define several and constant cycles of energy transformation, such as the rock cycle. However, in thermodynamics, the reversibility of natural processes only occurs when they do not lead to an increase in entropy. In this way, the cyclicity of geological processes does not show absolute stability, and transformations must be considered at an appropriate timescale. That is, both the planet's internal geodynamics and the complex astronomical system can be visualized as spiral cycles that constantly change at different timescales (e.g. Schwarzacher, 1993). This logical construction is similar to the one of perpetual movement of history proposed by Giambattista Vico, in which a new cycle always begins with a remnant of the cycle that ended (Vaughan, 1972).
It is challenging to think that the Earth itself is a specific product, in time and space, of the cyclic process of formation and destruction of stars, which has been repeated since the beginning of the Universe. Different chemical elements are formed at each new cycle and subtly change the star nebula composition resulting from the great supernova explosions. If it were not for the existence of one of these nebulae, with a particular chemical composition inherited from these past cycles, hovering in a specific corner of our galaxy 4.6 Ga ago, we would not have the Earth system as we know it today. Carl Sagan famously stated that “the cosmos is within us. We are made of star-stuff.” We are all, then, star-stuff on a deep journey of cyclic transformation.
No data sets were used in this article.
DGCF conceived the presented idea, wrote the manuscript draft, prepared the figures, and made changes to the manuscript according to the reviewers' suggestions. MK, AJCM, CMDSS, GPRG, and AS reviewed and improved the manuscript through corrections and suggestions.
The contact author has declared that neither they nor their co-authors have any competing interests.
Publisher's note: Copernicus Publications remains neutral with regard to jurisdictional claims in published maps and institutional affiliations.
The authors would like to thank Petrobras and the Petrobras School of High-Resolution Stratigraphy members. Special thanks to Dora Atman and Romeu Rossi Júnior for valuable discussions and suggestions. The authors are grateful to Andrew D. Miall and Annalisa Ferretti for detailed and constructive feedback as well as to editors Roman Leonhardt and Kristian Schlegel for their support during the review process. Daniel Galvão Carnier Fragoso and Matheus Kuchenbecker would like to make an honourable mention of their Alma Mater (Universidade Federal de Minas Gerais), especially Luiz Guilherme Knauer, Lúcia Maria Fantinel, and Ricardo Diniz da Costa.
This paper was edited by Roman Leonhardt and reviewed by Andrew Miall and Annalisa Ferretti.
Abels, H. A., Kraus, M. J., and Gingerich, P. D.: Precession-scale cyclicity in the fluvial lower Eocene Willwood Formation of the Bighorn Basin, Wyoming (USA), Sedimentology, 60, 1467–1483, https://doi.org/10.1111/sed.12039, 2013.
Adhémar: Révolutions de la Mer: Déluges périodiques, 440 pp.,1860.
Agassiz, L.: Études Sur Les Glaciers, Cambridge University Press, 363 pp., ISBN 9781108049764, 2012.
Ager, D. V.: The new catastrophism: the importance of the rare event in geological history, Cambridge University Press, Cambridge, 231 pp., ISBN 0521483581, 1993.
Allen, J. R. L.: Asymmetrical ripple marks and the origin of water-laid Cosets of Cross-Strata, Geol. J., 3, 187–236, https://doi.org/10.1002/gj.3350030201, 1962.
Barrell, J.: Rhythms and the measurements of geologic time, GSA Bulletin, 28, 745–904, https://doi.org/10.1130/GSAB-28-745, 1917.
Beckinsale, R. P. and Chorley, R. J.: The History of the Study of Landforms – Volume 3 (Routledge Revivals): Historical and Regional Geomorphology, 1890–1950, Taylor & Francis, ISBN 978-0415568012, 2003.
Beerbower, J. R.: Cyclothems and Cyclic Depositional Mechanisms in Alluvial Plain Sedimentation, in: Symposium on cyclic sedimentation, 169, edited by: Merriam, D. F., Kansas Geological Survey, Kansas Geological Survey, Bulletin 169, United States of America, 31–42, 1964.
Behdad, A.: A step toward the practical stratigraphic automatic correlation of well logs using continuous wavelet transform and dynamic time warping technique, J. Appl. Geophys., 167, 26–32, https://doi.org/10.1016/j.jappgeo.2019.05.007, 2019.
Bellucci, F., Woo, J., Kilburn, C. R., and Rolandi, G.: Ground deformation at Campi Flegrei, Italy: implications for hazard assessment, Geol. Soc. Lond. Sp. Publ., 269, 141–157, https://doi.org/10.1144/GSL.SP.2006.269.01.09, 2006.
Berger, A., Loutre, M. F., and Dehant, V.: Astronomical frequencies for pre-Quaternary palaeoclimate studies, Terra Nova, 1, 474–479, https://doi.org/10.1111/j.1365-3121.1989.tb00413.x, 1989.
Berger, A., Mesinger, F., and Sijacki, D.: Climate Change: Inferences from Paleoclimate and Regional Aspects, Springer Science & Business Media, 244 pp., 2012.
Bernard, H. A. and Major Jr., C. F.: Recent Meander Belt Deposits of the Brazos River: An Alluvial, AAPG Bull., 47, 350–350, 1963.
Boulila, S., Laskar, J., Haq, B. U., Galbrun, B., and Hara, N.: Long-term cyclicities in Phanerozoic sea-level sedimentary record and their potential drivers, Glob. Planet. Change, 165, 128–136, https://doi.org/10.1016/j.gloplacha.2018.03.004, 2018.
Boulila, S., Haq, B. U., Hara, N., Müller, R. D., Galbrun, B., and Charbonnier, G.: Potential encoding of coupling between Milankovitch forcing and Earth's interior processes in the Phanerozoic eustatic sea-level record, Earth-Sci. Rev., 220, 103727, https://doi.org/10.1016/j.earscirev.2021.103727, 2021.
Boutsikas, E. and Ruggles, C.: Temples, stars, and ritual landscapes: the potential for archaeoastronomy in ancient Greece, Am. J. Archaeol., 115, 55–68, https://doi.org/10.3764/aja.115.1.0055, 2011.
Brodzikowski, K. and van Loon, A. J.: Glacigenic Sediments, Elsevier Science, Amsterdam, 978-0-08-086963-6, 2014.
Cannatelli, C., Spera, F. J., Bodnar, R. J., Lima, A., and De Vivo, B.: Ground movement (bradyseism) in the Campi Flegrei volcanic area, in: Vesuvius, Campi Flegrei, and Campanian Volcanism, Elsevier, 407–433, https://doi.org/10.1016/B978-0-12-816454-9.00015-8, 2020.
Catuneanu, O.: Principles of sequence stratigraphy, 1st edn., Elsevier, Amsterdam; Boston, 375 pp., 2006.
Catuneanu, O.: Model-independent sequence stratigraphy, Earth-Sci. Rev., 188, 312–388, https://doi.org/10.1016/j.earscirev.2018.09.017, 2019a.
Catuneanu, O.: Scale in sequence stratigraphy, Mar. Petrol. Geol., 106, 128–159, https://doi.org/10.1016/j.marpetgeo.2019.04.026, 2019b.
Catuneanu, O. and Zecchin, M.: High-resolution sequence stratigraphy of clastic shelves II: Controls on sequence development, Mar. Petrol. Geol., 39, 26–38, https://doi.org/10.1016/j.marpetgeo.2012.08.010, 2013.
Catuneanu, O., Galloway, W. E., Kendall, C. G. S. t. C., Miall, A. D., Posamentier, H. W., Strasser, A., and Tucker, M. E.: Sequence Stratigraphy: Methodology and Nomenclature, nos, 44, 173–245, https://doi.org/10.1127/0078-0421/2011/0011, 2011.
Cecil, C. B.: The concept of autocyclic and allocyclic controls on sedimentation and stratigraphy, emphasizing the climatic variable, in: Climate Controls on Stratigraphy, SEPM (Society for Sedimentary Geology), Special Publication 77, edited by: Cecil, C. B. and Edgar, N. T., United States of America, 13–20, https://doi.org/10.2110/pec.03.77.0013, 2003.
Chamberlin, T. C.: The Ulterior Basis of Time Divisions and the Classification of Geologic History, J. Geol., 6, 449–462, https://doi.org/10.1086/608138, 1898.
Chamberlin, T. C.: Diastrophism as the ultimate basis of correlation, J. Geol., 17, 685–693, https://doi.org/10.1086/621676, 1909.
Chandler, B. M. P. and Evans, D. J. A.: Glacial Processes and Sediments, in: Encyclopedia of Geology, Elsevier, 830–856, https://doi.org/10.1016/B978-0-12-409548-9.11902-5, 2021.
Chorley, R. J., Dunn, A. J., and Beckinsale, R. P.: The History of the Study of Landforms, Vol. 1, Geomorphology Before Davis: Or the Development of Geomorphology, Routledge, 678 pp., 2009.
Clube, S. V. M. and Napier, W. M.: Galactic dark matter and terrestrial periodicities, Q. J. Roy. Astron. Soc., 37, 618–642, 1996.
Croll, J.: Climate and Time in Their Geological Relations: A Theory of Secular Changes of the Earth's Climate, D. Appleton, 624 pp., 1875.
Cross, T. A. and Homewood, P. W.: Amanz Gressly's role in founding modern stratigraphy, GSA Bull., 109, 1617–1630, https://doi.org/10.1130/0016-7606(1997)109<1617:AGSRIF>2.3.CO;2, 1997.
Davis, W. M.: The geographical cycle, Geogr. J., 14, 481–504, https://doi.org/10.2307/1774538, 1899.
Davis, W. M.: Peneplains and the geographical cycle, GSA Bull., 33, 587–598, https://doi.org/10.1130/GSAB-33-587, 1922.
Dott Jr., R. H.: Episodic sedimentation – how normal is average? How rare is rare? Does it matter?, J. Sediment. Petrol., 53, 5–23, https://doi.org/10.1306/212F8148-2B24-11D7-8648000102C1865D, 1983.
Dott Jr., R. H.: Chapter 1: An introduction to the ups and downs of eustasy, in: Eustasy: The Historical Ups and Downs of a Major Geological Concept, Vol. 180, edited by: Dott Jr., R. H., Geological Society of America, https://doi.org/10.1130/MEM180-p1, 1992.
Dott, R. H.: Laurence L. Sloss and the Sequence Stratigraphy Revolution, GSA Today, 24, 24–26, 2014.
Dutton, C. E.: ART. XI. – A Criticism upon the Contractional Hypothesis, Am. J. Sci. Art., 8, 113–123, https://doi.org/10.2475/ajs.s3-8.44.113, 1874.
Einsele, G.: Sedimentary basins: evolution, facies, and sediment budget, 2nd, completely rev. and enl. ed ed., Springer, Berlin, New York, 792 pp., 2000.
Emiliani, C.: Pleistocene temperatures, J. Geol., 63, 538–578, https://doi.org/10.1086/626295, 1955.
Emiliani, C.: Paleotemperature analysis of Caribbean cores P6304-8 and P6304-9 and a generalized temperature curve for the past 425,000 years, J. Geol., 74, 109–124, https://doi.org/10.1086/627150, 1966.
Emiliani, C., Hudson, J. H., Shinn, E. A., and George, R. Y.: Oxygen and carbon isotopic growth record in a reef coral from the Florida Keys and a deep-sea coral from Blake Plateau, Science, 202, 627–629, https://doi.org/10.1126/science.202.4368.627, 1978.
Engel, A. E. J. and Engle, C. B.: Continental accretion and the evolution of North America, in: Advancing Frontiers in Geology and Geophysics, edited by: Subramaniam, A. P. and Balakrishna, S., Indian Geophysical Union, Hyderabad, 17–37, 1964.
Esmark, J.: Bidrag til vor jordklodes historie, Magazin for Naturvidenskaberne, Anden Aargangs förste Bind, Förste Hefte, 3, 28–49, 1824.
Faria, D. L. de P., Tadeu dos Reis, A., and Gomes de Souza, O.: Three-dimensional stratigraphic-sedimentological forward modeling of an Aptian carbonate reservoir deposited during the sag stage in the Santos basin, Brazil, Mar. Petrol. Geol., 88, 676–695, https://doi.org/10.1016/j.marpetgeo.2017.09.013, 2017.
Ferretti, A., Vezzani, F., and Balini, M.: Leonardo da Vinci (1452–1519) and the birth of stratigraphy, Newsl. Stratigr., 53, 1–17, https://doi.org/10.1127/nos/2019/0564, 2020.
Fischer, A. G.: The Lofer cyclothem of the Alpine Triassic, in: Symposium on cyclic sedimentation, 169, edited by: Merriam, D. F., Kansas Geological Survey, United States of America, 107–149, 1964.
Fischer, A. G.: Climatic oscillations in the bioshere, in: Biotic Crises in Ecological and Evolutionary Time, edited by: Nitecki, M. H., Academic Press, 103–131, https://doi.org/10.1016/B978-0-12-519640-6.50012-0, 1981.
Fischer, A. G.: Long-term climatic oscillations recorded in Stratigraphy, in: Climate in Earth History, National Academies Press, Washington, 97–105, https://doi.org/10.17226/11798, 1982.
Fisher, W. L., Gama Jr., E., and Ojeda, H. A. O.: Estratigrafia sísmica e sistemas deposicionais da Formação Piaçabuçu, XXVII, Congresso Brasileiro de Geologia, Aracaju, 123–134, 1973.
Fisk, H. N., Kolb, C. R., McFarlan, E., and Wilbert, L. J.: Sedimentary framework of the modern Mississippi delta [Louisiana], J. Sediment. Res., 24, 76–99, https://doi.org/10.1306/D4269661-2B26-11D7-8648000102C1865D, 1954.
Fragoso, D. G. C., Gabaglia, G. P. R., Magalhães, A. J. C., and Scherer, C. M. dos S.: Cyclicity and hierarchy in sequence stratigraphy: an integrated approach, Braz. J. Geol., 51, e20200106, https://doi.org/10.1590/2317-4889202120200106, 2021.
Frodeman, R.: Geological reasoning: Geology as an interpretive and historical science, GSA Bull., 107, 960–968, https://doi.org/10.1130/0016-7606(1995)107<0960:GRGAAI>2.3.CO;2, 1995.
Frodeman R.: Hermeneutics in the Field: The Philosophy of Geology, in: The Multidimensionality of Hermeneutic Phenomenology, Contributions to Phenomenology, edited by: Babich, B. and Ginev, D., Springer, 69–79, https://doi.org/10.1007/978-3-319-01707-, 2014.
Gilbert, G. K.: Lake Bonneville, Lake Bonneville, U.S. Government Printing Office, Washington, DC, https://doi.org/10.3133/m1, 1890.
Gilbert, G. K.: Sedimentary Measurement of Cretaceous Time, J. Geol., 3, 121–127, https://doi.org/10.1086/607150, 1895.
Glennie, K. W.: Desert sedimentary environments, Elsevier, ISBN 9780080869254, 2010.
Gnibidenko, H. S. and Shashkin, K. S.: Basic principles of the geosynclinal theory, Tectonophysics, 9, 5–13, https://doi.org/10.1016/0040-1951(70)90025-9, 1970.
Goldhammer, R. K.: Cyclic sedimentation, in: Sedimentology, Springer Berlin Heidelberg, Berlin, Heidelberg, 271–293, https://doi.org/10.1007/3-540-31079-7_57, 1978.
Grabau, A.: Oscillation or pulsation, 16th International Geological Congress, Washington, Report, 539–552, 1936.
Gradstein, F. M., Ogg, J. G., Schmitz, M. D., and Ogg, G. M.: Geologic Time Scale 2020, Elsevier, ISBN 9780128243619, 2020.
Gregor, B.: Some ideas on the rock cycle: 1788–1988, Geochim. Cosmochim. Ac., 56, 2993–3000, https://doi.org/10.1016/0016-7037(92)90285-Q, 1992.
Gressly, A.: Observations géologiques sur le Jura soleurois, Petitpierre, Imprimerie de Petitpierre, 349 pp., 1838.
Hajek, E. A. and Straub, K. M.: Autogenic Sedimentation in Clastic Stratigraphy, Annu. Rev. Earth Planet. Sc., 45, 681–709, https://doi.org/10.1146/annurev-earth-063016-015935, 2017.
Hallam, A.: Secular changes in marine inundation of USSR and North America through the Phanerozoic, Nature, 269, 769–772, https://doi.org/10.1038/269769a0, 1977.
Haq, B. U. and Schutter, S. R.: A chronology of Paleozoic sea-level changes, Science, 322, 64–68, https://doi.org/10.1126/science.1161648, 2008.
Hawkesworth, C. J. and Brown, M.: Earth dynamics and the development of plate tectonics, The Royal Society Publishing, https://doi.org/10.1098/rsta.2018.0228, 2018.
Hawkins, G. S.: Stonehenge Decoded, Nature, 200, 306–308, https://doi.org/10.1038/200306a0, 1963.
Hays, J. D., Imbrie, J., and Shackleton, N. J.: Variations in the Earth's Orbit: Pacemaker of the Ice Ages: For 500,000 years, major climatic changes have followed variations in obliquity and precession, Science, 194, 1121–1132, https://doi.org/10.1126/science.194.4270.1121, 1976.
Hestmark, G.: Jens Esmark's mountain glacier traverse 1823 – the key to his discovery of Ice Ages, Boreas, 47, 1–10, https://doi.org/10.1111/bor.12260, 2017.
Hilgen, F., Schwarzacher, W., and Strasser, A.: Concept and Definitions in Cyclostratigraphy (Second Report of the Cyclostratigraphy Working Group): International Subcommission on Stratigraphic Nomenclature of the IUGS Commission on Stratigraphy, in: Cyclostratigraphy: Approaches and Case Histories, vol. 81, edited by: D'Argenio, B., Fischer, A. G., Premoli Silva, I., Weissert, H., and Ferreri, V., SEPM Soc. Sediment. Geol., 81, 303–305, https://doi.org/10.2110/pec.04.81.0303, 2004.
Hinnov, L. A.: Cyclostratigraphy and astrochronology in 2018, in: Stratigraphy & Timescales, Vol. 3, Elsevier, 1–80, https://doi.org/10.1016/bs.sats.2018.08.004, 2018.
Hinnov, L. A. and Park, J.: Detection of astronomical cycles in the stratigraphic record by frequency modulation (FM) analysis, J. Sediment. Res., 68, 524–539, https://doi.org/10.2110/jsr.68.524, 1998.
Hockey, T., Trimble, V., Williams, T. R., Bracher, K., Jarrell, R. A., Marché, J. D., Palmeri, J., and Green, D. W. E. (Eds.): Biographical Encyclopedia of Astronomers, Springer New York, New York, NY, https://doi.org/10.1007/978-1-4419-9917-7, 2014.
Holbrook, J. M. and Miall, A. D.: Time in the Rock: A field guide to interpreting past events and processes from siliciclastic stratigraphy, Earth-Sci. Rev., 203, 103121, https://doi.org/10.1016/j.earscirev.2020.103121, 2020.
House, M. R.: Orbital forcing timescales: an introduction, Geol. Soc. Lond. Sp. Publ., 85, 1–18, https://doi.org/10.1144/GSL.SP.1995.085.01.01, 1995.
Huang, X., Griffiths, C. M., and Liu, J.: Recent development in stratigraphic forward modelling and its application in petroleum exploration, Austr. J. Earth Sci., 62, 903–919, https://doi.org/10.1080/08120099.2015.1125389, 2015.
Hunt, D. and Tucker, M. E.: Stranded parasequences and the forced regressive wedge systems tract: deposition during base-level'fall, Sediment. Geol., 81, 1–9, https://doi.org/10.1016/0037-0738(92)90052-S, 1992.
Husinec, A., Basch, D., Rose, B., and Read, J. F.: FISCHERPLOTS: An Excel spreadsheet for computing Fischer plots of accommodation change in cyclic carbonate successions in both the time and depth domains, Comput. Geosci., 34, 269–277, https://doi.org/10.1016/j.cageo.2007.02.004, 2008.
Illing, L. V.: Bahaman calcareous sands, AAPG Bull., 38, 1–95, https://doi.org/10.1306/5CEADEB4-16BB-11D7-8645000102C1865D, 1954.
Imbrie, J. and Imbrie, K. P.: Ice ages: solving the mystery, Harvard University Press, ISBN 0674440757, 1986.
Jamieson, T. F.: On the History of the Last Geological Changes in Scotland, Q. J. Geol. Soc., 21, 161–204, https://doi.org/10.1144/GSL.JGS.1865.021.01-02.24, 1865.
Johnson, M. E.: Chap. 5, A. W. Grabau's embryonic sequence stratigraphy and eustatic curve, in: Geological Society of America Memoirs, Vol. 180, Geological Society of America, 43–54, https://doi.org/10.1130/MEM180-p43, 1992.
Karato, S. and Barbot, S.: Dynamics of fault motion and the origin of contrasting tectonic style between Earth and Venus, Sci. Rep., 8, 1–11, https://doi.org/10.1038/s41598-018-30174-6, 2018.
Kearey, P., Klepeis, K. A., and Vine, F. J.: Global tectonics, John Wiley & Sons, ISBN 978-1-405-10777-8, 2009.
Klein, G. deV and Willard, D. A.: Origin of the Pennsylvanian coal-bearing cyclothems of North America, Geology, 17, 152–155, https://doi.org/10.1130/0091-7613(1989)017<0152:OOTPCB>2.3.CO;2, 1989.
Kodama, K. P. and Hinnov, L. A.: Rock magnetic cyclostratigraphy, Wiley-Blackwell, Chichester, West Sussex, UK, ISBN 978-1-118-56128-7, 2015.
Kravitz, G.: The Geohistorical Time Arrow: From Steno's Stratigraphic Principles to Boltzmann's Past Hypothesis, J. Geosci. Educ., 62, 691–700, https://doi.org/10.5408/13-107.1, 2014.
Krumbein, W. C. and Dacey, M. F.: Markov chains and embedded Markov chains in geology, Mathemat. Geol., 1, 79–96, https://doi.org/10.1007/BF02047072, 1969.
Kvale, E. P.: Tides and tidal rhytmites, in: Sedimentology, Springer Berlin Heidelberg, Berlin, Heidelberg, 1224–1228, https://doi.org/10.1007/3-540-31079-7_238, 1978.
Laskar, J., Fienga, A., Gastineau, M., and Manche, H.: La2010: a new orbital solution for the long-term motion of the Earth, Astron. Astrophys., 532, A89, https://doi.org/10.1051/0004-6361/201116836, 2011.
Le Pichon, X.: Fifty years of plate tectonics: Afterthoughts of a witness, Tectonics, 38, 2919–2933, https://doi.org/10.1029/2018TC005350, 2019.
Li, M., Huang, C., Ogg, J., Zhang, Y., Hinnov, L., Wu, H., Chen, Z.-Q., and Zou, Z.: Paleoclimate proxies for cyclostratigraphy: Comparative analysis using a Lower Triassic marine section in South China, Earth-Sci. Rev., 189, 125–146, https://doi.org/10.1016/j.earscirev.2019.01.011, 2019.
Lima, A., De Vivo, B., Spera, F. J., Bodnar, R. J., Milia, A., Nunziata, C., Belkin, H. E., and Cannatelli, C.: Thermodynamic model for uplift and deflation episodes (bradyseism) associated with magmatic–hydrothermal activity at the Campi Flegrei (Italy), Earth-Sci. Rev., 97, 44–58, https://doi.org/10.1016/j.earscirev.2009.10.001, 2009.
Lirer, F. and Iaccarino, S.: Mediterranean Neogene historical stratotype sections and Global Stratotype Section and Point (GSSP): state of the art, Ann. Naturhist. Mus. Wien Ser. A, 113, 67–144, 2011.
Lyell, C.: Principles of geology, John Murray, 1835.
Maclaren, C.: The glacial Theory of Prof. Agassiz, Am. J. Sci. Art., 42, 346–365, 1842.
Magalhães, A. J. C., Raja Gabaglia, G. P., Scherer, C. M. S., Bállico, M. B., Guadagnin, F., Bento Freire, E., Silva Born, L. R., and Catuneanu, O.: Sequence hierarchy in a Mesoproterozoic interior sag basin: from basin fill to reservoir scale, the Tombador Formation, Chapada Diamantina Basin, Brazil, Basin Res., 28, 393–432, https://doi.org/10.1111/bre.12117, 2016.
Magalhães, A. J. C., Lima-Filho, F. P., Guadagnin, F., Silva, V. A., Teixeira, W. L. E., Souza, A. M., Raja Gabaglia, G. P., and Catuneanu, O.: Ground penetrating radar for facies architecture and high-resolution stratigraphy: Examples from the Mesoproterozoic in the Chapada Diamantina Basin, Brazil, Mar. Petrol. Geol., 86, 1191–1206, https://doi.org/10.1016/j.marpetgeo.2017.07.027, 2017.
Magalhães, A. J. C., Raja Gabaglia, G. P., Fragoso, D. G. C., Bento Freire, E., Lykawka, R., Arregui, C. D., Silveira, M. M. L., Carpio, K. M. T., De Gasperi, A., Pedrinha, S., Artagão, V. M., Terra, G. J. S., Bunevich, R. B., Roemers-Oliveira, E., Gomes, J. P., Hernández, J. I., Hernández, R. M., and Bruhn, C. H. L.: High-resolution sequence stratigraphy applied to reservoir zonation and characterisation, and its impact on production performance – shallow marine, fluvial downstream, and lacustrine carbonate settings, Earth-Sci. Rev., 210, 103325, https://doi.org/10.1016/j.earscirev.2020.103325, 2020.
Martinez, M., Kotov, S., De Vleeschouwer, D., Pas, D., and Pälike, H.: Testing the impact of stratigraphic uncertainty on spectral analyses of sedimentary series, Clim. Past, 12, 1765–1783, https://doi.org/10.5194/cp-12-1765-2016, 2016.
Maslin, M.: Forty years of linking orbits to ice ages, Nature, 540, 208–209, https://doi.org/10.1038/540208a, 2016.
Matenco, L. C. and Haq, B. U.: Multi-scale depositional successions in tectonic settings, Earth-Sci. Rev., 200, 102991, https://doi.org/10.1016/j.earscirev.2019.102991, 2020.
Mazur, A.: Amadeus Grabau in China: 1920–1946, Carbonate. Evaporite., 21, 51–93, https://doi.org/10.1007/BF03175468, 2006.
Miall, A. D.: Empiricism and model building in stratigraphy: the historical roots of present-day practices, Stratigraphy, 1, 3–25, 2004.
Miall, A.D.: Updating uniformitarianism: stratigraphy as just a set of “frozen accidents”, Geological Society of London, Special Publications, 404, 11–36, https://doi.org/10.1144/SP404.4, 2015.
Miall, A. D.: Stratigraphy: A Modern Synthesis, Springer International Publishing, Cham, https://doi.org/10.1007/978-3-319-24304-7, 2016.
Miall, A. D., Holbrook, J. M., Bhattacharya, J. P.: The Stratigraphy Machine, J. Sediment. Res., 91, 595–610, https://doi.org/10.2110/jsr.2020.143, 2021.
Miall, A. D. and Miall, C. E.: Empiricism and model-building in stratigraphy: around the hermeneutic circle in the pursuit of stratigraphic correlation, Stratigraphy, 1, 27–46, 2004.
Middleton, G. V. (Ed.): Primary Sedimentary Structures and their Hydrodynamic Interpretation, SEPM Spec. Publ.,12, 265 pp., 1965.
Middleton, G. V.: Johannes Walther's Law of the Correlation of Facies, GSA Bull., 84, 979–988, https://doi.org/10.1130/0016-7606(1973)84<979:JWLOTC>2.0.CO;2, 1973.
Middleton, G. V.: Sedimentary geology, in: Sedimentology, Springer Netherlands, Dordrecht, https://doi.org/10.1007/3-540-31079-7_184, 1978.
Milankovitch, M.: Kanon der Erdbestrahlung und seine Anwendung auf das Eiszeitenproblem, Mihaila Ćurčića, Belgrade, 633 pp., 1941.
Mitchell, R. N., Spencer, C. J., Kirscher, U., He, X.-F., Murphy, J. B., Li, Z.-X., and Collins, W. J.: Harmonic hierarchy of mantle and lithospheric convective cycles: Time series analysis of hafnium isotopes of zircon, Gondwana Res., 75, 239–248, https://doi.org/10.1016/j.gr.2019.06.003, 2019.
Mitchum Jr., R. M.: Seismic stratigraphy and global changes of sea level: Part 11. Glossary of terms used in seismic stratigraphy: Section 2. Application of seismic reflection configuration to stratigraphic interpretation, in: Seismic Stratigraphy: Applications to Hydrocarbon Exploration, edited by: Payton, C. E., AAPG Memoir, 26, 51–52, 1977.
Mitchum Jr., R. M. and Vail, P. R.: Seismic stratigraphy and global changes of sea level: Part 7. Seismic stratigraphic interpretation procedure: Section 2. Application of seismic reflection configuration to stratigraphic interpretation, in: Seismic Stratigraphy: Applications to Hydrocarbon Exploration, edited by: Payton, C. E., AAPG Memoir, 26, 135–143, 1977.
Moore, R. C.: Stratigraphic classification of the Pennsylvanian rocks of Kansas, Kansas Geological Survey Bulletin, Tulsa, 22, 256 pp., 1936.
Moore, R. C.: Paleoecological aspects of Kansas Pennsylvanian and Permian cyclothems, in: Symposium on cyclic sedimentation, 169, edited by: Merriam, D. F., Kansas Geological Survey, United States of America, 287–380, 1964.
Müller, R. D. and Dutkiewicz, A.: Oceanic crustal carbon cycle drives 26-million-year atmospheric carbon dioxide periodicities, Sci. Adv., 6, eaaq0500, https://doi.org/10.1126/sciadv.abd0953, 2018.
Montañez, I., Norris, R., MA, C., Johnson, K., MJ, K., Kiehl, J., Kump, L., Ravelo, A., and KK, T.: Understanding Earth's Deep Past: Lessons for our Climate Future, The National Academies Press, Washington, DC, ISBN 978-0-309-20919-9, 2011.
Nelson, H.: Kykloi: cyclic theories in ancient Greece, M.S, Portland State University, United States of America, https://doi.org/10.15760/etd.3256, 1980.
Nagel, E.: The Structure of Science: Problems in the Logic of Scientific Explanation, Harcourt, Brace & World, United States of America, ISBN 0710018827, 1961.
Nio, S. D., Brouwer, J. H., Smith, D., de Jong, M., and Böhm, A. R.: Spectral trend attribute analysis: applications in the stratigraphic analysis of wireline logs, First Break, 23, 71–75, https://doi.org/10.3997/1365-2397.23.4.26503, 2005.
O'Hara, K. D.: A Brief History of Geology, Cambridge University Press, Cambridge, United Kingdom, https://doi.org/10.1017/9781316809990, 2018.
Oomkens, E. and Terwindt, J. H. J.: Inshore estuarine sediments in the Haringvliet (Netherlands), Geologie en mijnbouw : orgaan voor officieele mededelingen van het Geologisch-Mijnbouwkundig Genootschap voor Nederland en Kolonien, 39, 701–710, 1960.
Paillard, D.: Glacial cycles: toward a new paradigm, Rev. Geophys., 39, 325–346, https://doi.org/10.1029/2000RG000091, 2001.
Pantopoulos, G., Vakalas, I., Maravelis, A., and Zelilidis, A.: Statistical analysis of turbidite bed thickness patterns from the Alpine fold and thrust belt of western and southeastern Greece, Sediment. Geol., 294, 37–57, https://doi.org/10.1016/j.sedgeo.2013.05.007, 2013.
Parascandola, A. (Ed.): I fenomeni bradisismici del Serapeo di Pozzuoli, Stabilmento tipografico G. Genovese, 117 pp., 1947.
Payton, C. E. (Ed.): Seismic Stratigraphy — Applications to Hydrocarbon Exploration, American Association of Petroleum Geologists, 516 pp., https://doi.org/10.1306/M26490, 1977.
Posarnentier, H. W. and Allen, G. P. (Eds.): Siliciclastic Sequence Stratigraphy, SEPM (Society for Sedimentary Geology), SEPM (Society for Sedimentary Geology), United States of America, https://doi.org/10.2110/csp.99.07, 1999.
Peloggia, A. U. G.: The Rock Cycle of the Anthropocene: inserting human agency into the Earth System, Revista do Instituto Geológico, 39, 1–13, https://doi.org/10.5935/0100-929x.20180001, 2018.
Posamentier, H. W. and Allen, G. P.: Siliciclastic sequence stratigraphy, Concepts and Applications: SEPM, Concepts in Sedimentology and Paleontology, 7, 210 pp., https://doi.org/10.2110/csp.99.07, 1999.
Posamentier, H. W., Jervey, M. T., and Vail, P. R.: Eustatic Controls on Clastic Deposition I—Conceptual Framework, in: Sea-Level Changes: An Integrated Approach, vol. 42, edited by: Wilgus, C. K., Hastings, B. S., Posamentier, H., Wagoner, J. V., Ross, C. A., and Kendall, C. G. St. C., SEPM Society for Sedimentary Geology, 109-124, https://doi.org/10.2110/pec.88.01.0109, 1988.
Preston, F. W. and Henderson, J.: Fourier series characterization of cyclic sediments for stratigraphic correlation, in: Symposium on cyclic sedimentation, 169, edited by: Merriam, D. F., Kansas Geological Survey, United States of America, 415–425, 1964.
Puche-Riart, O.: History of Geology up to 1780, in: Encyclopedia of Geology, Elsevier, 167–172, https://doi.org/10.1016/B0-12-369396-9/00367-1, 2005.
Puetz, S. J.: The Unified Cycle Theory: How Cycles Dominate the Structure of the Universe and Influence Life on Earth, Outskirts Press, United States of America, 489 pp., ISBN 9781432712167, 2009.
Purkis, S., Vlaswinkel, B., and Gracias, N.: Vertical-To-Lateral Transitions Among Cretaceous Carbonate Facies–A Means To 3-D Framework Construction Via Markov Analysis, J. Sediment. Res., 82, 232–243, https://doi.org/10.2110/jsr.2012.23, 2012.
Rampino, M. R., Caldeira, K., and Zhu, Y.: A pulse of the Earth: A 27.5-Myr underlying cycle in coordinated geological events over the last 260 Myr, Geosci. Front., 12, 101245, https://doi.org/10.1016/j.gsf.2021.101245, 2021.
Randall, L. and Reece, M.: Dark Matter as a Trigger for Periodic Comet Impacts, Phys. Rev. Lett., 112, 1–5, https://doi.org/10.1103/PhysRevLett.112.161301, 2014.
Read, J. F. and Goldhammer, R. K.: Use of Fischer plots to define third-order sea-level curves in Ordovician peritidal cyclic carbonates, Appalachians, Geology, 16, 895–899, https://doi.org/10.1130/0091-7613(1988)016<0895:UOFPTD>2.3.CO;2, 1988.
Rich, J. L.: Three critical environments of deposition, and criteria for recognition of rocks deposited in each of them, Geol. Soc. Am. Bull., 62, 1–20, https://doi.org/10.1130/0016-7606(1951)62[1:TCEODA]2.0.CO;2, 1951.
Richet, P., Henderson, G., and Neuville, D.: Thermodynamics: The Oldest Branch of Earth Science?, Element, 6, 287–292, https://doi.org/10.2113/gselements.6.5.287, 2010.
Sadler, P.: The influence of hiatuses on sediment accumulation rates, in on the determination of sediment accumulation rates, GeoResearch Forum, 5, 5–40, 1999.
Sames, B., Wagreich, M., Conrad, C. P., and Iqbal, S.: Aquifer-eustasy as the main driver of short-term sea-level fluctuations during Cretaceous hothouse climate phases, Geological Society, London, Special Publications, 498, 9–38, https://doi.org/10.1144/SP498-2019-105, 2020.
Schulz, M. and Schäfer-Neth, C.: Translating Milankovitch climate forcing into eustatic fluctuations via thermal deep water expansion: a conceptual link, Terra Nova, 9, 228-231, https://doi.org/10.1111/j.1365-3121.1997.tb00018.x, 1998.
Schwarzacher, W.: Sedimentation models and quantitative stratigraphy, Elsevier, the Netherlands, 381 pp., ISBN 9780080869315, 1975.
Schwarzacher, W.: Cyclostratigraphy and the Milankovitch Theorym, Elsevier, the Netherlands, 226 pp., ISBN 9780080869667, 1993.
Schwarzacher, W.: Repetitions and cycles in stratigraphy, Earth-Sci. Rev., 50, 51–75, https://doi.org/10.1016/S0012-8252(99)00070-7, 2000.
Seranne, M.: Early Oligocene stratigraphic turnover on the west Africa continental margin: a signature of the Tertiary greenhouse-to-icehouse transition?, Terra Nova, 11, 135–140, https://doi.org/10.1046/j.1365-3121.1999.00246.x, 1999.
Shackleton, N.: Oxygen isotope analyses and Pleistocene temperatures re-assessed, Nature, 215, 15–17, https://doi.org/10.1038/215015a0, 1967.
Shaviv, N. J., Prokoph, A., and Veizer, J.: Is the solar system's galactic motion imprinted in the Phanerozoic climate?, Sci. Rep., 4, 1–6, https://doi.org/10.1038/srep06150, 2014.
Shearman, D. J.: Origin of marine evaporites by diagenesis, Transactions of the Institute of Mining and Metallurgy, Section B, 75, 208–215, 1966.
Shi, B., Chang, X., Liu, Z., Pang, Y., Xu, Y., Mao, L., Zhang, P., and Chen, G.: Intelligent identification of sequence stratigraphy constrained by multipopulation genetic algorithm and dynamic time warping technique: A case study of Lower Cretaceous Qingshuihe Formation in hinterland of Junggar Basin (NW China), Basin Res., 33, 2517–2544, https://doi.org/10.1111/bre.12567, 2021.
Simons, D. B. and Richardson, E. V.: Resistance to flow in alluvial channels, Professional Paper, U. S. Govt. Print. Off., 70 pp., https://doi.org/10.3133/pp422J, 1966.
Sloss, L. L.: Sequences in the Cratonic Interior of North America, GSA Bull., 74, 93–114, https://doi.org/10.1130/0016-7606(1963)74[93:SITCIO]2.0.CO;2, 1963.
Sloss, L. L., Krumbein, W. C., and Dapples, E. C.: Integrated Facies Analysis, in: Geological Society of America Memoirs, 39, Geol. Soc. Am., 39, 91–124, https://doi.org/10.1130/MEM39-p91, 1949.
Stern, R. J. and Scholl, D. W.: Yin and yang of continental crust creation and destruction by plate tectonic processes, Int. Geol. Rev., 52, 1–31, https://doi.org/10.1080/00206810903332322, 2010.
Stille, H.: Grundfragen der vergleichenden Tektonik, Nature, 117, 192–192, https://doi.org/10.1038/117192b0, 1926.
Strasser, A.: Hiatuses and condensation: an estimation of time lost on a shallow carbonate platform, Depositional Record, 1, 91–117, https://doi.org/10.1002/dep2.9, 2015.
Strasser, A.: Cyclostratigraphy of shallow-marine carbonates–limitations and opportunities, in: Cyclostratigraphy and Astrochronology, 3, edited by: Michael Montenari, Elsevier, the Netherlands, 151–187, https://doi.org/10.1016/bs.sats.2018.07.001, 2018.
Strasser, A., Pittet, B., Hillgärtner, H., and Pasquier, J.-B.: Depositional sequences in shallow carbonate-dominated sedimentary systems: concepts for a high-resolution analysis, Sediment. Geol., 128, 201–221, https://doi.org/10.1016/S0037-0738(99)00070-6, 1999.
Strasser, A., Hilgen, F. J., and Heckel, P. H.: Cyclostratigraphy-concepts, definitions, and applications, Newsl. Stratigr., 42, 75–114, https://doi.org/10.1127/0078-0421/2006/0042-0075, 2006.
Suess, E.: Das antlitz der erde, F. Tempsky, 1888.
Teichert, C.: Concepts of Facies, AAPG Bull., 42, 2718–2744, https://doi.org/10.1306/0BDA5C0C-16BD-11D7-8645000102C1865D, 1958.
Timothy, R. C.: Log-Linear Models, Markov Chains and Cyclic Sedimentation, SEPM JSR, 52, 905–912, https://doi.org/10.1306/212F808A-2B24-11D7-8648000102C1865D, 1982.
Udden, J. A.: Geology and mineral resources of the Peoria quadrangle, Illinois, Bull. Govt. Print. Off., 506, 103 pp., https://doi.org/10.3133/b506, 1912.
Vail, P. R.: Chap. 8, The evolution of seismic stratigraphy and the global sea-level curve, in: Eustasy: The Historical Ups and Downs of a Major Geological Concept, edited by: Dott Jr., R. H., Geological Society of America, 180, 83–92, https://doi.org/10.1130/MEM180-p83, 1992.
Vail, P. R., Mitchum, R. M., and Thompson, S.: Seismic Stratigraphy and Global Changes of Sea Level, Part 4: Global Cycles of Relative Changes of Sea Level, in: Seismic Stratigraphy: Applications to Hydrocarbon Exploration, edited by: Payton, C. E., AAPG Memoir, 26, 83–97, https://doi.org/10.1306/M26490C6, 1977.
Van Houten, F. B.: Cyclic lacustrine sedimentation, upper Triassic Lockatong formation, central New Jersey and adjacent Pennsylvania, in: Symposium on cyclic sedimentation, 169, edited by: Merriam, D. F., Kansas Geological Survey, United States of America, 497–531, 1964.
Van Siclen, D. C.: Depositional topography – examples and theory, AAPG Bull., 42, 1897–1913, https://doi.org/10.1306/0BDA5B88-16BD-11D7-8645000102C1865D, 1958.
Van Wagoner, J. C., Mitchum, R. M., Campion, K. M., and Rahmanian, V. D.: Siliciclastic sequence stratigraphy in well logs, cores, and outcrops: concepts for high-resolution correlation of time and facies, American Association of Petroleum Geologists, United States of America, https://doi.org/10.1306/Mth7510, 1990.
Vaughan, F.: The Political Philosophy of Giambattista Vico: An Introduction to La Scienza Nuova, Springer, the Netherlands, https://doi.org/10.1007/978-94-010-2781-6, 1972.
Wagreich, M., Sames, B., Hart, M., and Yilmaz, I. O.: An introduction to causes and consequences of Cretaceous sea-level changes (IGCP 609), Geol. Soc. Lond. Sp. Publ., 498, 1–8, https://doi.org/10.1144/SP498-2019-156, 2020.
Walker, J. D., Geissman, J. W., Bowring, S. A., and Babcock, L. E.: The Geological Society of America geologic time scale, GSA Bull., 125, 259–272, https://doi.org/10.1130/B30712.1, 2013.
Wanless, H. R.: Geology and Mineral Resources of the Alexis Quadrangle, Illinois State Geological Survey Bulletin, 57, United States of America, 268 pp., ISBN 10 0265850355, 1929.
Wanless, H. R.: Pennsylvanian Section in Western Illinois, GSA Bull., 42, 801–812, https://doi.org/10.1130/GSAB-42-801, 1931.
Wanless, H. R. and Weller, J. M.: Correlation and Extent of Pennsylvanian Cyclothems, GSA Bull., 43, 1003–1016, https://doi.org/10.1130/GSAB-43-1003, 1932.
Weedon, G. P.: Time-Series Analysis and Cyclostratigraphy: Examining Stratigraphic Records of Environmental Cycles, 1st edn., Cambridge University Press, https://doi.org/10.1017/CBO9780511535482, 2003.
Wegener, A.: Die Entstehung der Kontinente und Ozeane, Braunschweig, 94, ISBN 9783443010560, 1915.
Weller, J. M.: Cyclical Sedimentation of the Pennsylvanian Period and Its Significance, J. Geol., 38, 97–135, https://doi.org/10.1086/623695, 1930.
Weller, J. M.: Rhythms in upper Pennsylvanian cyclothems, Illinois State Geological Survey Bulletin, 92, United States of America, 1943.
Weller, J. M.: Cyclothems and larger sedimentary cycles of the Pennsylvanian, J. Geol., 66, 195–207, https://doi.org/10.1086/626494, 1958.
Weller, J. M.: Stratigraphic principles and practice, Harper, United States of America, 725 pp., 1960.
Weller, J. M.: Development of the concept and interpretation of cyclic sedimentation, in: Symposium on cyclic sedimentation, 169, edited by: D.F. Merriam, Kansas Geological Survey, United States of America, 607–621, 1964.
Westerhold, T., Marwan, N., Drury, A. J., Liebrand, D., Agnini, C., Anagnostou, E., Barnet, J. S., Bohaty, S. M., De Vleeschouwer, D., and Florindo, F.: An astronomically dated record of Earth's climate and its predictability over the last 66 million years, Science, 369, 1383–1387, https://doi.org/10.1126/science.aba6853, 2020.
Wilgus, C. K., Hastings, B. S., Posamentier, H., Wagoner, J. V., Ross, C. A., and Kendall, C. G. St. C. (Eds.): Sea-Level Changes: An Integrated Approach, SEPM Society for Sedimentary Geology, 407 pp., 1988.
Willis, B.: Principles of paleogeography, Science, 31, 241–260, https://doi.org/10.1126/science.31.790.241, 1910.
Wilson, J. T.: A new class of faults and their bearing on continental drift, Nature, 207, 343–347, https://doi.org/10.1038/207343a0, 1965.
Wilson, J. T.: Did the Atlantic close and then re-open?, Nature, 211, 676–681, https://doi.org/10.1038/211676a0, 1966.
Wilson, R. C. L.: Sequence stratigraphy: a revolution without a cause?, Geol. Soc. Lond. Sp. Publ., 143, 303–314, https://doi.org/10.1144/GSL.SP.1998.143.01.20, 1998.
Wilson, R. W., Houseman, G. A., Buiter, S. J. H., McCaffrey, K. J., and Doré, A. G.: Fifty years of the Wilson Cycle concept in plate tectonics: an overview, Geol. Soc. Lond. Sp. Publ., 470, 1–17, https://doi.org/10.1144/SP470-2019-58, 2019.
Zeller, E.J., Cycles and psychology, in: Symposium on cyclic sedimentation, 169, edited by: Merriam, D. F., Kansas Geological Survey, United States of America, 631–636, 1964.